《认知心理学》课程教学资源(书籍文献)聋人听觉皮层在感知觉功能重组中的作用 Reorganization of large-scale brain networks in deaf signing adults - The role of auditory cortex in functional reorganization following deafness
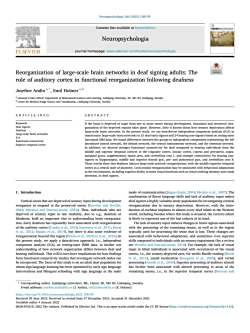
Contents lists available at Neuropsychologia ELSEVIER journal homepage:www.elsevier.com/locate/neuropsychologia Reorganization of large-scale brain networks in deaf signing adults:The role of auditory cortex in functional reorganization following deafness Josefine Andin,Emil Holmer. ARTICLE INFO ABSTRACT vity for deaf supp nge as a central 1.Introduction Cortical areas that are deprived of input during development cortical )Thus,individuals who are e current c is likely to repre d,20 sing the sen s lost.Thes e changes a am to individualswithno ation differs betw nput in blind individuals is ass ted with rec ment of the visua e and ized by of山 interventions and bilingual schooling with sign language as the m remaining senses,i.e.,in the superior temporal cortex (Stevens and 028392/e202m0X202 s.Published by Elsevier Ltd.This is an open access article under the CC BY license (http://crear org/licemses/by/4.0/)
Neuropsychologia 166 (2022) 108139 Available online 4 January 2022 0028-3932/© 2022 The Authors. Published by Elsevier Ltd. This is an open access article under the CC BY license (http://creativecommons.org/licenses/by/4.0/). Reorganization of large-scale brain networks in deaf signing adults: The role of auditory cortex in functional reorganization following deafness Josefine Andin a,* , Emil Holmer a,b a Linnaeus Centre HEAD, Department of Behavioural Sciences and Learning, Linkoping ¨ University, SE, 581 83, Link¨ oping, Sweden b Center for Medical Image Science and Visualization, Link¨ oping University, Sweden ARTICLE INFO Keywords: Deaf signers Deafness Large-scale brain networks ICA Functional connectivity Superior temporal cortex ABSTRACT If the brain is deprived of input from one or more senses during development, functional and structural reorganization of the deprived regions takes place. However, little is known about how sensory deprivation affects large-scale brain networks. In the present study, we use data-driven independent component analysis (ICA) to characterize large-scale brain networks in 15 deaf early signers and 24 hearing non-signers based on resting-state functional MRI data. We found differences between the groups in independent components representing the left lateralized control network, the default network, the ventral somatomotor network, and the attention network. In addition, we showed stronger functional connectivity for deaf compared to hearing individuals from the middle and superior temporal cortices to the cingulate cortex, insular cortex, cuneus and precuneus, supramarginal gyrus, supplementary motor area, and cerebellum crus 1, and stronger connectivity for hearing nonsigners to hippocampus, middle and superior frontal gyri, pre- and postcentral gyri, and cerebellum crus 8. These results show that deafness induces large-scale network reorganization, with the middle/superior temporal cortex as a central node of plasticity. Cross-modal reorganization may be associated with behavioral adaptations to the environment, including superior ability in some visual functions such as visual working memory and visual attention, in deaf signers. 1. Introduction Cortical areas that are deprived of sensory input during development reorganize to respond to the preserved senses (Bavelier and Neville, 2002; Merabet and Pascual-Leone, 2010). Thus, individuals who are deprived of sensory input in one modality, due to e.g., deafness or blindness, hold an important clue to understanding brain reorganization. Early deafness has repeatedly been associated with reorganization of the auditory cortex (Cardin et al., 2018; Emmorey et al., 2011; Karns et al., 2012; Malaia et al., 2014), but there is also some evidence of reorganization beyond this region (Bonna et al., 2020; Li et al., 2016). In the present study, we apply a data-driven approach, i.e., independent component analysis (ICA) on resting-state fMRI data, to further our understanding of how network organization differs between deaf and hearing individuals. This will in turn have implications for how findings from functional connectivity studies that investigate network nodes can be interpreted. The focus of this study is on an adult deaf population for whom sign language learning has been optimized by early sign language interventions and bilingual schooling with sign language as the main mode of communication (Bagga-Gupta, 2004; Meristo et al., 2007). The combination of fluent language skills and lack of auditory input makes deaf signers a highly valuable study population for investigating cortical reorganization due to sensory deprivation. However, with the introduction of cochlear implants in almost every deaf infant in the Western world, including Sweden where this study is situated, the current cohort is likely to represent one of the last cohorts of its kind. The lack of sensory input induces changes in brain regions associated with the processing of the remaining senses, as well as in the region typically used for processing the sense that is lost. These changes are associated with behavioral adaptations, and sometimes even superior skills compared to individuals with no sensory impairment (for a review see Merabet and Pascual-Leone, 2010). For example, the lack of visual input in blind individuals is associated with recruitment of the visual cortex, i.e., the sensory-deprived area, for tactile Braille reading (Reich et al., 2011), sound localization (Gougoux et al., 2005), and verbal processing (Amedi et al., 2004). Superior processing of auditory stimuli has further been associated with altered processing in areas of the remaining senses, i.e., in the superior temporal cortex (Stevens and * Corresponding author. Linkopings ¨ universitet, IBL, I-huset, SE, 581 83, Linkoping, ¨ Sweden. E-mail addresses: josefine.andin@liu.se (J. Andin), emil.holmer@liu.se (E. Holmer). Contents lists available at ScienceDirect Neuropsychologia journal homepage: www.elsevier.com/locate/neuropsychologia https://doi.org/10.1016/j.neuropsychologia.2021.108139 Received 30 June 2021; Received in revised form 17 December 2021; Accepted 31 December 2021
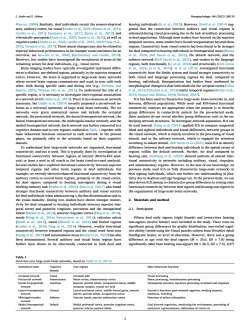
J.Andin and E Holmer mp chologin166(2022)10813 Ding et al as ork (Dell Duc t al.2 nd weaker to the lan eeriortempor ctivityto urther s Ye d the role of a 2012) poralegonsGHri e)m on ne ICA has 2018sS ks in network,a ork separation,ICA ca ether s bet paper we primarily refer to the networks based on their cognitive u,and in the salience ne ork.which is ale and h ring d.This is typi with he defa brain (seed voxel analy ctivity in orks includ erize larg x to eral brain region s,prim ly in the visual ue to eafness and sign langu euse.I e present study nal co ivity betw he organization ot large.scale brain network 2.Materials and method 2.1.Participants insula (Ding et al 2016 Amit et al. Fifteen deaf early signers(eight female)and tw enty-four hearing ere n et a 2018:Dinz et al 2016).H to be Table 1 works,based on icta
Neuropsychologia 166 (2022) 108139 2 Weaver, 2009). Similarly, deaf individuals recruit the sensory-deprived area, auditory cortex, for visual (Andin et al., 2021; Bottari et al., 2014; Cardin et al., 2013; Emmorey et al., 2011; Karns et al., 2012) and vibrotactile perception (Auer et al., 2007; Karns et al., 2012), as well as cognitive tasks (Andin et al., 2021; Cardin et al., 2013, 2018; Ding et al., 2015; Twomey et al., 2017). These neural changes may also be related to superior behavioral performances in for example visual attention (for an overview, see Bavelier et al., 2006; MacSweeney and Cardin, 2015). However, few studies have investigated the recruitment of areas of the remaining senses for deaf individuals, e.g., visual cortex. Brain imaging studies have typically investigated task-based differences in distinct, pre-defined regions, primarily in the superior temporal cortex. However, the brain is organized in large-scale brain networks where several brain regions communicate and work in sync with each other, both during specific tasks and during rest (e.g., Petersen and Sporns, 2015; Thomas Yeo et al., 2011). To understand the role of a specific region, it is necessary to investigate inter-regional associations at a network level (Uddin et al., 2019). There is no consensus on network taxonomy, but Uddin et al. (2019) recently proposed a six-network solution as a universal taxonomy of large scale brain networks. The six networks were given anatomical names and include the occipital network, the pericentral network, the dorsal frontoparietal network, the lateral frontoparietal network, the midcingulo-insular network, and the medial frontoparietal network. Each network was further connected to a cognitive domain and to core regions, outlined in Table 1 together with main behavioral functions connected to each network. In the present paper, we primarily refer to the networks based on their cognitive domain. To understand how large-scale networks are organized, functional connectivity analysis is used. This is typically done by investigation of functional connectivity between regions of interest (ROI-to-ROI analysis) or from a seed to all voxels in the brain (seed-to-voxel analysis). Several studies have explored functional connectivity between superior temporal regions and other brain regions in deaf individuals. For example, we recently showed enhanced functional connectivity from the auditory cortex to several brain regions, primarily in the visual cortex, for deaf signers compared to hearing non-signers during a visual working memory task (Andin et al., 2021). Bola et al. (2017) also found stronger functional connectivity between auditory and visual cortices for deaf individuals when administering a rhythm discrimination task in the visual modality. During rest, studies have shown stronger connectivity for deaf compared to hearing individuals between superior temporal cortex and posterior cingulate, precuneus and the intraparietal lobule (Malaia et al., 2014), anterior cingulate cortex (Ding et al., 2016), insula (Ding et al., 2016; Striem-Amit et al., 2016), calcarine sulcus (Shiell et al., 2014), visual (Benetti et al., 2021) and frontal regions (Cardin et al., 2018; Ding et al., 2016). However, weaker functional connectivity between temporal regions and the visual word form area (Wang et al., 2015) and somatomotor areas (Bonna et al., 2020) has also been demonstrated. Several auditory and visual brain regions have further been shown to be structurally connected in both deaf and hearing individuals (Li et al., 2015). However, Shiell et al. (2014) suggested that the connection between auditory and visual regions is enhanced during visual processing due to the lack of auditory processing in deaf populations. Although most studies have focused on the superior temporal cortices, some studies have found reorganization beyond these regions. Connectivity from visual cortices has been found to be stronger for deaf compared to hearing individuals to frontoparietal areas (Bonna et al., 2020; Dell Ducas et al., 2021), the default network, and the salience network (Dell Ducas et al., 2021), and weaker to the language regions, both functionally (Li et al., 2016) and structurally (Dell Ducas et al., 2021; Li et al., 2015). Li et al. (2016) investigated functional connectivity from the limbic system and found stronger connectivity to both visual and language processing regions for deaf, compared to hearing, individuals. Reorganization has further been confirmed by morphological changes in deaf individuals for the occipital cortex (Allen et al., 2013; P´enicaud et al., 2012) and in temporal regions (Hribar et al., 2014; Kumar and Mishra, 2018; Shibata, 2007). ICA has been used to characterize large-scale brain networks in, and between, different populations. While seed- and ROI-based functional connectivity analyses are appropriate when the purpose is to describe group differences in connectivity pattern for the pre-selected seeds, these analyses do not reveal whether group differences exist in the underlying network structures. To investigate network separation, ICA can be used instead. Wang et al. (2014) applied ICA to compare congenital blind and sighted individuals and found differences between groups in the visual network, which is mainly involved in the processing of visual stimuli, and in the salience network, which is engaged for attention switching to salient stimuli. Dell Ducas et al. (2021) used ICA to identify difference between deaf and hearing individuals in the spatial extent of regions within the default network. Further, for deaf compared to hearing cats, Stolzberg et al. (2018) showed patterns of altered functional connectivity in networks including auditory, visual, cingulate, and somatosensory regions. However, to the best of our knowledge, no previous study used ICA to fully characterize large-scale networks in deaf signing individuals, which can further our understanding of plasticity due to deafness and sign language use. In the present study, we use data-driven ICA aiming to characterize group differences in resting-state functional connectivity between deaf signers and hearing non-signers in the organization of large-scale brain networks. 2. Materials and method 2.1. Participants Fifteen deaf early signers (eight female) and twenty-four hearing non-signers (twelve female) were included in the study. There were no significant group differences for gender distribution, non-verbal cognitive ability (tested using the Visual puzzles subtest from Weschler Adult Intelligence Scale), or level of education. However, there was a group difference in age with the deaf signers (M = 35.0, SD = 7.8) being significantly older than hearing non-signers (M = 26.5, SD = 7.5), t(37) Table 1 Overview over large-scale brain networks, based on Uddin et al. (2019). Anatomical name Cognitive domain Core regions Main behavioral functions Occipital network Visual Occipital lobe Visual processing Pericentral network Somatomotor Motor cortex, somatosensory cortex Motor processing, somatosensory processing Dorsal frontoparietal network Attention Superior parietal lobule, intraparietal sulcus, middle temporal complex, frontal eye field Visuospatial attention; top-down processing of stimuli and responses Lateral frontoparietal network Control Lateral prefrontal cortex, middle frontal gyrus, anterior inferior parietal lobule, intraparietal sulcus Executive functions; goal-oriented cognition, working memory, inhibition, switching Midcingulo-insular network Salience Anterior insula, anterior midcinulate cortex Detection of salient information Medial frontoparietal network Default Medial prefrontal cortex, posterior cingulate cortex, posterior inferior parietal lobule Goal directed cognitvion, monitoring the environment, processing of associative representations, elaboration of events etc J. Andin and E. Holmer
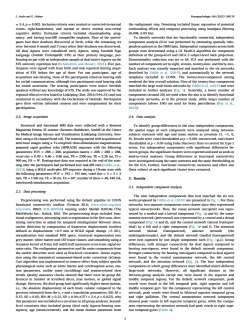
eropsycholegin 166 (2022)10813 A. =0.002.1 visio right ess.and normal or abow nommal non-ve non-MBn preg og。5 a (0 Nine of the vorks that are functionally nnected.independer had their end ye aining si erTminecdu5ingaCCfaiCAaotnorf 0 npone ent sample of sion ing acqu ach a cted and matched to the six netw singNone of the par er cluded in CONN.The venty-four. hed the larg 019)and wer oard in Linkopi d by the uded in arge 01 2.2.Image acquisition 2.4.Data analysis Structural and functional MRI data collected with a Si es in the nine inde b) the spatial mapso each component were analyzed u 10 D).Re were voxe 1atp<0.001 incorrecte preparedrapidgad t echo (MPRAGE)seg th rrors.For in ent com 090×086×086mTR2300m 236 analyses Grou ou tas-E rus (ndin (beta values)of each significant cluster were extracted mm,TR- 69,number of slices =48.440 vol. 3.Results 3.1.Independent component analysis 2.3.Data processing The nine inde et al (201 ched the six ne nn,RRID: unning under Matlab R2018 orks. the visual netw repr irst sc represented by d a d pn of f e disp nt (o etal)by a nd arightcom and )The atten ization into standard MNI s ral seg inte sular). and the default network (me dial fro ( atio.The tound in th nt-based on (a the ventral soma the left co physi Oualit wed that the e no o dif e found in the sup n had s or gan nd in the pole,right brain volu 0.05;HN SD 0.04:37) 4,p 0.023 why es thus include (deaf ear anng nt rer network had peak voxels in right sup
Neuropsychologia 166 (2022) 108139 3 = 3.4, p = 0.002. Inclusion criteria were normal or corrected-to-normal vision, right-handedness, and normal or above normal non-verbal cognitive ability. Exclusion criteria included claustrophobia, pregnancy, and having non-MR compatible implants. Nine of the participants had their deafness discovered at birth, while the remaining six were between 6 month and 3 years when their deafness was discovered. All deaf signers were considered early signers, using Swedish Sign Language (Svenskt Teckenspråk; STS) as their primary language, performing on par with an independent sample of deaf native signers on the STS sentence repetition test (Schonstr ¨ om ¨ and Hauser, 2021). Five participants were signed with from birth and nine reported starting acquisition of STS before the age of three. For one participant, age of acquisition was missing. None of the participants relied on hearing aids for verbal communication, although two participants used hearing aids for sound awareness. The hearing participants were native Swedish speakers without any knowledge of STS. The study was approved by the regional ethical review board in Linkoping ¨ (Dnr, 2016/344–31) and was conducted in accordance with the Declaration of Helsinki. Participants gave their written informed consent and were compensated for their participation. 2.2. Image acquisition Structural and functional MRI data were collected with a Siemens Magnetom Prisma 3T scanner (Siemens Healthcare, GmbH) at the Center for Medical Image Science and Visualization (Linko¨ping University, Sweden) using a 64-channel head coil. The scanning started with acquisition of structural images using a T1-weighted three-dimensional magnetizationprepared rapid gradient echo (MPRAGE) sequence with the following parameters: FOV = 288 × 288, acquisition matrix = 208 × 288 × 288, voxel size = 0.90 × 0.86 × 0.86 mm, TR = 2300 ms, TE = 2.36 ms, TI = 900 ms, FA = 8◦. Resting-state data was acquired at the end of the scanning after the participants had performed four task-EPI runs (Andin et al., 2021), using a BOLD multi-plex EPI sequence during a 10-min scan with the following parameters: FOV = 192 × 192 mm, voxel size = 3 × 3 × 3 mm, TR = 1340 ms, TE = 30 ms, FA = 69◦, number of slices = 48, 440 vol, interleaved/simultaneous acquisition. 2.3. Data processing Preprocessing was performed using the default pipeline in CONN functional connectivity toolbox (Version 20.b; www.nitric.org/proj ects/conn, RRID: SCR_009550) running under Matlab R2018a (The MathWorks Inc., Natick, MA). The preprocessing steps included functional realignment, unwarping and co-registration to the first scan, slicetiming correction to adjust for temporal misalignment between slices, outlier detection by computation of framewise displacement (outliers defined as displacement >0.9 mm or BOLD signal change >5 SD.), normalization into standard MNI space, structural segmentation into grey matter, white matter and CSF tissue classes, and smoothing using a Gaussian kernel of 8 mm full width half maximum to increase signal-tonoise ratio. The realignment parameters and the noise components from the outlier detection were used as first-level covariates. Linear regression using the anatomical component-based noise correction (aCompCor) algorithm was implemented to remove effect from subject specific physiological noise such as white matter and cerebrospinal areas, motion parameters, outlier scans (scrubbing) and session-related slow trends. Quality assurance checks showed that there were no group differences in number of scrubbed slices, max motion, or global signal change. However, the deaf group had significantly higher mean motion, i.e., the absolute displacement of each brain volume compared to the previous estimated from the x, y and z translation parameters (DS: M = 0.15, SD = 0.05; HN: M = 0.12, SD = 0.04; t(37) = 2.4, p = 0.023), why this parameter was included as a covariate in all group analyses. Secondlevel covariates thus included group (deaf early signers/hearing nonsigners), age (mean-centered), and the mean motion parameter from the realignment step. Denoising included linear regression of potential confounding effects and temporal processing using bandpass filtering (0.008, 0.09 Hz). To identify networks that are functionally connected, independent component analysis (ICA) was performed by estimating spatially independent patterns in the fMRI data. Independent components across both groups were determined using a G1 FastICA algorithm for component definition at the group-level and GICA 3 subject-level back projection. Dimensionality reduction was set to 64. ICA was performed with the number of components set to eight, sixteen, twenty-four, and thirty-two. Each analysis was visually inspected and matched to the six networks described by Uddin et al. (2019), and automatically to the network templates included in CONN. The twenty-four-component setting rendered the best overall solution. Nine of the twenty-four components matched the large scale brain networks by Uddin et al. (2019) and were included in further analyses (Fig. 1). Generally, a lower number of components (around 20) are used when the aim is to identify functional large-scale networks, as in the present study, while larger number of components (above 100) are used for brain parcellation (Ray et al., 2013). 2.4. Data analysis To identify group-differences in the nine independent components, the spatial maps of each component were analyzed using betweensubjects contrasts with age and mean motion as covariate (1, − 1, 0, 0). Results were voxel thresholded at p < 0.001 uncorrected, and cluster thresholded at p < 0.05 using False Discovery Rate to control for type 1 errors. For independent components with significant differences between groups, the significant clusters were exported and used as seeds in seed-to-voxel analyses. Group differences in functional connectivity were investigated using the same contrasts and the same thresholding as for the ICA. Further, functional connectivity measures and effect size (beta values) of each significant cluster were extracted. 3. Results 3.1. Independent component analysis The nine independent components that best matched the six networks proposed by Uddin et al. (2019) are presented in Fig. 1. For three networks, two separate components were chosen since they represented typical sub-networks. Thus, the visual network (occipital) was represented by a medial and a lateral component (Fig. 1a and b), the somatomotor network (pericentral) was represented by a ventral and a dorsal component (Fig. 1c and d), and the control network (lateral frontoparietal) by a left and a right component (Fig. 1e and f). The attention network (dorsal frontoparietal), salience network (the midcingulo-insular), and the default network (medial frontoparietal) were best captured by one single component each (Fig. 1g–i). Group differences, with stronger connectivity for deaf signers compared to hearing non-signers, were found in the default network component. Stronger connectivity for hearing non-signers compared to deaf signers were found in the ventral somatomotor network, the left control network, and the attention network (Fig. 2). The four independent components in which group differences were identified match different large-scale networks. However, all significant clusters in the between-group analysis except one were found in the superior and middle temporal regions. For the default network component, peak voxels were found in the left temporal pole, right superior and left middle temporal gyri. For the component representing the left control network, peak voxels were found in bilateral superior temporal gyrus and right pallidum. The ventral somatomotor network component showed peak voxels in left superior temporal gyrus, while the component representing the attention network had peak voxels in right superior temporal gyrus (Table 2). J. Andin and E. Holmer
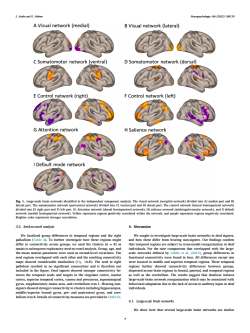
J.Andin and E Holme Neuropsycholegin 166 (2022)108139 A Visual network(medial) B Visual network(lateral) C Somatomotor network(ventral) D Somatomotor network(dorsal) E Control network(right) F Control network(left) G Attention network H Salience network A2 Default mode network ork (occipital network)divided into a)medial part and B ght part and F)e k)di fdo (mi urple repr Brighter color represents stronger correla 3.2.Seed-to-voxel analysi 4.Discussion s in deaf si ers.Our findings onfim analysis.Gr or the nir nts that o pped with the larg ed with each other nd the r found in four.all diffe except on hese tempor d in in the parietal, ior temp corte cun us and prect upram arge ed strong individuals 4.1 arge-scle brain network We show here that several large-cale brain networks are simila
Neuropsychologia 166 (2022) 108139 4 3.2. Seed-to-voxel analysis We localized group differences in temporal regions and the right palladium (Table 2). To further investigate how these regions might differ in connectivity across groups, we used the clusters (n = 8) as masks in subsequent exploratory seed-to-voxel analysis. Group, age, and the mean motion parameter were used as second-level covariates. The seed regions overlapped with each other and the resulting connectivity maps showed considerable similarities (Fig. 3A-E). The seed in right pallidum resulted in no significant connections and is therefore not included in the figure. Deaf signers showed stronger connectivity between the temporal seeds and targets in the cingulate cortex, insular cortex, superior temporal cortex, cuneus and precuneus, supramarginal gyrus, supplementary motor area, and cerebellum crus 1. Hearing nonsigners showed stronger connectivity to clusters including hippocampus, middle/superior frontal gyrus, pre- and postcentral gyrus, and cerebellum crus 8. Details of connectivity measures are provided in Table S1. 4. Discussion We sought to investigate large-scale brain networks in deaf signers, and how these differ from hearing non-signers. Our findings confirm that temporal regions are subject to cross-modal reorganization in deaf individuals. For the nine components that overlapped with the largescale networks defined by Uddin et al. (2019), group differences in functional connectivity were found in four. All differences except one were located in middle and superior temporal regions. These temporal regions further showed connectivity differences between groups, dispersed across brain regions in frontal, parietal, and temporal regions as well as the cerebellum. The results suggest that deafness induces large-scale brain network reorganization which may be associated with behavioral adaptation due to the lack of access to auditory input in deaf individuals. 4.1. Large-scale brain networks We show here that several large-scale brain networks are similar Fig. 1. Large-scale brain networks identified in the independent component analysis. The visual network (occipital network) divided into A) medial part and B) lateral part. The somatomotor network (pericentral network) divided into C) ventral part and D) dorsal part. The control network (lateral frontoparietal network) divided into E) right part and F) left part. G) Attention network (dorsal frontoparietal network), H) salience network (midcingulo-insular network), and I) default network (medial frontoparietal network). Yellow represents regions positively correlated within the network, and purple represents regions negatively correlated. Brighter color represents stronger correlation. J. Andin and E. Holmer
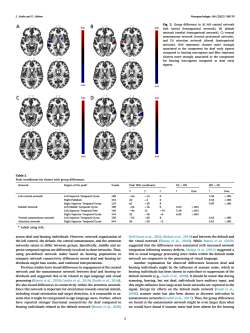
J.Andin and E Holm DS HN Left control network ral Gyrus Default network 39445 46 Labels using AAL netwo divid cptnatioforobecrgddifteg ces between d ng (B net al.. 20 okda et al.2015) ing.he we
Neuropsychologia 166 (2022) 108139 5 across deaf and hearing individuals. However, network organization of the left control, the default, the ventral somatomotor, and the attention networks seems to differ between groups. Specifically, middle and superior temporal regions are differently involved in these networks. Thus, using pre-defined network nodes based on hearing populations to compare network connectivity differences across deaf and hearing individuals might bias results, and confound interpretations. Previous studies have found differences in engagement of the control network and the somatomotor network between deaf and hearing individuals and suggested this to be related to sign language and visual processing (Bonna et al., 2020; Cardin et al., 2018; Okada et al., 2016). We also found differences in connectivity within the attention network. Since this network is important for orientation towards external stimuli, including visual orientation and target detection, it is reasonable to assume that it might be reorganized in sign language users. Further, others have reported stronger functional connectivity for deaf compared to hearing individuals related to the default network (Bonna et al., 2020; Dell Ducas et al., 2021; Malaia et al., 2014) and between the default and the visual network (Bonna et al., 2020). While Bonna et al. (2020) suggested that the differences were associated with increased network integration following sensory deficits, Malaia et al. (2014) suggested a link to visual language processing since nodes within the default mode network are responsive to the processing of visual language. Another explanation for observed differences between deaf and hearing individuals might be the influence of scanner noise, which in hearing individuals has been shown to contribute to suppression of the default network (e.g., Gaab et al., 2008). It should be noted that during scanning, hearing, but not deaf, individuals have auditory input, and this might influence how large-scale brain networks are captured in the signal. Except for effects on the default mode network (Gaab et al., 2008), scanner noise has also been shown to decrease activation in somatomotor networks (Andoh et al., 2017). Thus, the group differences we found in the somatomotor network might be even larger than what we would have found if scanner noise had been absent for the hearing Fig. 2. Group difference in A) left control network (left lateral frontoparietal network), B) default network (medial frontoparietal network), C) ventral somatomotor network (ventral pericentral network), and D) attention network (dorsal frontoparietal network). Red represents clusters more strongly associated to the component for deaf early signers compared to hearing non-signers and Blue represent clusters more strongly associated to the component for hearing non-signers compared to deaf early signers. Table 2 Peak coordinates for cluster with group differences. Network Region of the peaka Voxels Peak MNI coordinates DS > HN HN > DS x y z t pFDR t pFDR Left control network Left Superior Temporal Gyrus 189 − 56 − 14 0 4.55 <.001 Right Pallidum 164 22 − 2 6 5.52 <.001 Right Superior Temporal Gyrus 124 62 − 20 8 4.87 <.001 Default network Left Middle Temporal Gyrus 189 − 56 − 16 0 5.63 <.001 Left Superior Temporal Pole 146 − 46 12 − 14 5.38 <.001 Right Superior Temporal Gyrus 144 52 − 18 − 6 6.05 <.001 Ventral somatomotor network Left Superior Temporal Gyrus 150 − 52 − 20 8 4.63 <.001 Attention network Right Superior Temporal Gyrus 544 56 − 20 − 2 5.61 <.001 a Labels using AAL. J. Andin and E. Holmer
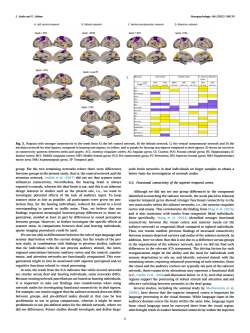
J.Andin and E Holmer Neuropsycholegin 166 (2022)108139 group.For the twe emaining networks where there were diff ot see that scar as the deaf brain is not.and this is an nen uperiortempoalgyrushovedtromgerfnctionalconmetiytoth or funet &e compatort nity be spaWearenotabtetodiierentiaiebetwent由eroleofsignlangy group hat sumun,the inte ant ICA component The driving factors for suc tor,and at a y on.and identify.ex mal stimuli with the pen nal proces deaf individua orks differ in 4 consideralion8t the and attention and aic ork nodes 1o ey et a engroups,and pre-defined nodes should in that be les ar in in the visul domain.While langt e must be c unct
Neuropsychologia 166 (2022) 108139 6 group. For the two remaining networks where there were differences between groups in the present study, that is, the control network and the attention network, Andoh et al. (2017) did not see that scanner noise influences connectivity. Nevertheless, the hearing brain is always exposed to sounds, whereas the deaf brain is not, and this is an inherent design features in studies such as the present one, i.e., we want to investigate potential effects of the lack of auditory input. To keep scanner noise as low as possible, all participants were given ear protection that, for the hearing individuals, reduced the sound to a level corresponding to speech or traffic noise. Thus, we believe that our findings represent meaningful between-group differences in brain organization, molded at least in part by differences in sound perception between groups. However, to further investigate the specific effects of scanner noise in comparisons between deaf and hearing individuals, sparse imaging procedures could be used. We are not able to differentiate between the role of sign language and sensory deprivation with the current design, but the results of the present study, in combination with findings in previous studies, indicate that for individuals who do not process auditory stimuli, the interregional associations between auditory cortex and the control, somatomotor, and attention networks are functionally reorganized. This reorganization might in turn be associated with superior perceptual and/or cognitive functions related to visual processing. In sum, the result from the ICA indicates that while several networks are similar across deaf and hearing individuals, some networks differ. Because existing network parcellations are based on hearing individuals, it is important to take our findings into consideration when using network nodes for investigating functional connectivity in deaf signers. For example, our results suggest that the salience network does not differ between groups, and pre-defined nodes should in that case be less problematic to use in group comparisons, whereas it might be more problematic to use pre-defined nodes for the default network, where we did see differences. Future studies should investigate and define largescale brain networks in deaf individuals on larger samples to obtain a better basis for investigation of network nodes. 4.2. Functional connectivity of the superior temporal cortex Although we did not see any group differences in the component identified as matching the salience network, the seeds placed in bilateral superior temporal gyrus showed stronger functional connectivity to the two main nodes within the salience networks, i.e., the anterior cingulate cortex and insula. This corroborates the finding from Ding et al. (2016) and is also consistent with results from congenital blind individuals. More specifically, Wang et al. (2014) identified stronger functional connectivity between the visual cortex and anterior insula (of the salience network) in congenital blind compared to sighted individuals. Thus, our results confirm previous findings of increased connectivity between sensory-deprived cortices and nodes of the salience network. In addition, here we show that this is not due to a difference across groups in the organization of the salience network, since we did not find such differences in the relevant ICA component. The driving factors for such reorganization might be the ability and the need for individuals with sensory deprivation to rely on, and identify, external stimuli with the remaining senses, requiring enhanced processing of such stimulus. Since the visual and the auditory cortices are typically not part of the salience network, these connectivity alterations may represent a functional shift (c.f., Cardin et al., 2020 and discussion below, in 4.3), such that sensory regions support the processing of salient stimuli and attention and aid effective switching between networks in the deaf group. Several studies, including the seminal study by MacSweeney et al. (2006), have shown that the superior temporal cortex is important for language processing in the visual domain. While language input in the auditory domain enters the brain within the same lobe, language input from visual language must be conveyed there from the visual region, which might result in weaker functional connectivity within the superior Fig. 3. Regions with stronger connectivity to the seeds from A) the left control network, B) the default network, C) the ventral somatomotor network and D) the attention network for deaf signers, compared to hearing non-signers, in yellow, and in purple for hearing non-signers compared to deaf signers. E) shows an overview of connectivity patterns between seeds and targets. ACC Anterior cingulate cortex; AG Angular gyrus; CU Cuneus; FOG Frontal orbital gyrus; HC Hippocampus; IC Insular cortex; MCC Middle cingulate cortex; MFG Middle frontal gyrus; PCG Pre-/postcentral gyrus; PC Precuneus; SFG Superior frontal gyrus; SMA Supplementary motor area; SMG Supramarginal gyrus; TP Temporal pole. J. Andin and E. Holmer
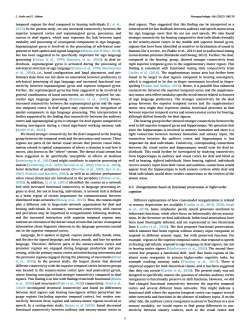
166 (2022)10813 he n the and h mot ity and p y-motor nd Wil 201) ing (Co t al. d to the hearing,group,showed stronger fro ng is con isten ity tion b d to be larg rin deaf sign een su s has be vity bet on of hand m 6 xand the the dea 1 deaf signers may re the might thus represent similar functie ted by n th s and hin us.iry be 21 mpus is ir ed in me rs are int in the pe bsent sense with Reorga ation based on functional preservation or higher-order on ation phasize purely neural pro unrelated o de Tthedorsalrth itera e on d ation about anguage processes carriec n et al 20】.The first or signed nds. to diff input,but with p d function.F fore differen of th d to sign in deaf si onal shift. that he ng of mov highe ogniti fo vity with the superi emp Icortex betv group d it ha 4:t had dea r the prese et al and st tural (Finkl et a 2020)co nectivity.Finkl eta tivity b dif an lar upeno emporal tex), in the a f on th 6peechaiestnagta e study,B (2020)found deer d expect to in co
Neuropsychologia 166 (2022) 108139 7 temporal regions for deaf compared to hearing individuals (Li et al., 2013). In the present study, we saw increased connectivity between the superior temporal cortex and supramarginal gyrus, precuneus, and cuneus in deaf signers, which may represent the link between input modality and processing of visuospatial components in sign language. Supramarginal gyrus is involved in the processing of sub-lexical components in both spoken and signed language (Malaia and Wilbur, 2010), but has been suggested to be specifically important for sign language processing (Corina et al., 1999; Emmorey et al., 2002). In deaf individuals, supramarginal gyrus is activated during the processing of sub-lexical structure of sign language (Corina et al., 1999; MacSweeney et al., 2008), i.e., hand configuration and hand placement, and preliminary data from our lab show an association between proficiency in sub-lexical processing of sign language and increased functional connectivity between supramarginal gyrus and superior temporal gyrus. Further, the supramarginal gyrus has been suggested to be involved in general coordination of hand movements, an ability required for successful sign language processing (Emmorey et al., 2002). Hence, increased connectivity between the supramarginal gyrus and the superior temporal cortex in deaf signers may represent the integration of spatial components in sign language processing. This interpretation is further supported by the finding that connectivity between the auditory cortex and supramarginal gyrus is stronger for deaf signers compared to hearing non-signers during a visual sign-based working memory task (Andin et al., 2021). We found stronger connectivity for the deaf compared to the hearing group between the temporal seeds and the precuneus and cuneus. These regions are parts of the dorsal visual stream that process visual information related to spatial components of where a stimulus is and how it moves, also known as the “where”-stream. The dorsal visual stream has been suggested to be specifically susceptible to effects of deafness (Armstrong et al., 2002) and might contribute to superior processing of motion (Armstrong et al., 2002; Bavelier et al., 2001; Fine et al., 2005), faster detection of peripheral events (Bavelier et al., 2001; Dye et al., 2007; Proksch and Bavelier, 2002), as well as to inferior performance when visual distractors are introduced in the periphery (Holmer et al., 2020). In addition, Li et al. (2016) identified the cuneus as a network hub with increased functional connectivity to language processing regions in deaf, but not in hearing, individuals. A network hub is defined as a brain region of certain importance for communication between distributed brain networks (Hwang et al., 2013). Thus, the cuneus might play a different role in large-scale network organization for deaf and hearing individuals. In summary, increased connectivity with cuneus and precuneus may be important in reorganization following deafness, and the increased interaction with superior temporal regions may indicate an extension of the dorsal visual stream that carries visuospatial information about linguistic elements to the language processes carried out in the superior temporal cortex. Language, be it spoken or signed, require motor skills; hands, arms, and the face for signed language, and throat, mouth, and face for spoken language. Therefore, different parts of the sensory-motor cortex and premotor regions are engaged during language production and processing, with the primary motor cortex activated during movements and the premotor regions engaged during the planning of movements (Finkl et al., 2020). In the present study, the largest cluster that showed different connectivity with the superior temporal cortex between groups was located in the sensory-motor cortex (pre- and postcentral gyrus), where hearing non-signers had stronger connectivity compared to deaf signers. This finding is in line with findings from both functional (Bonna et al., 2020) and structural (Finkl et al., 2020) connectivity. Finkl et al. (2020) investigated structural connectivity and found no differences between deaf signers and hearing non-signers within perisylvian language regions (including superior temporal cortex), but weaker connectivity between these regions and sensory-motor regions involved in speech. In a resting-state study, Bonna et al. (2020) found decreased functional connectivity between auditory and sensory-motor cortex in deaf signers. They suggested that this finding can be interpreted as a reduced need for fast feedback between auditory and speech motor areas for sign language users that do not use oral speech. We also found stronger connectivity for hearing compared to deaf individuals frontally to the sensory-motor cortex in the middle and superior frontal gyrus, regions that have been identified as sensitive to localization of sound in humans (for a review, see Plakke et al., 2014) and to audiovisual timing in non-human primates (Romanski and Hwang, 2012). Further, the deaf, compared to the hearing, group, showed stronger connectivity from right superior temporal gyrus to the supplementary motor region. This finding is consistent with functional resting state connectivity from Cardin et al. (2018). The supplementary motor area has further been found to be larger in deaf signers compared to hearing non-signers, which is suggested to be due to finger movements involved in fingerspelling (Kumar and Mishra, 2018). Hence, it is possible that enhanced connectivity between the superior temporal cortex and the supplementary motor area reflect modality-specific involvement related to features of sign language proficiency. The stronger connectivity for the deaf group between the superior temporal cortex and the supplementary motor area might thus represent similar functional processes as that between superior temporal cortex and sensory-motor cortex for hearing, although shifted frontally for deaf signers. The hearing group further showed stronger connectivity between the seed in left superior temporal gyrus and hippocampus. We speculate that since the hippocampus is involved in memory formation and there is a tight connection between memory formation and sensory input, the connection between the auditory cortex and hippocampus is less important for deaf individuals. Tentatively, corresponding connections between the visual cortex and hippocampus would exist for deaf individuals. This could further be investigated by studying connectivity from hippocampus to auditory and visual cortex for deaf and blind as well as hearing, sighted individuals. Since hearing, sighted, individuals receive sensory input from both vision and audition, connections would appear from the hippocampus to both sensory cortices while deaf and blind individuals would show weaker connections to the cortices of the absent sense. 4.3. Reorganization based on functional preservation or higher-order cognition Different explanations of how cross-modal reorganization is related to sensory deprivation are available (Cardin et al., 2018, 2020; Singh et al., 2018). Some emphasize purely neural processes unrelated to behavioral functions, while other focus on behaviorally driven associations. In the literature on deaf individuals, behavioral associations have been most thoroughly debated, and are represented in two theoretical lines (Cardin et al., 2020). The first proposes functional preservation, which assumes that brain regions without sensory input reorganize to respond to different sensory input, but with preserved function. For example, regions in the superior temporal cortex, that respond to speech in hearing individuals, respond to sign language in deaf signers, but not in hearing native signers (MacSweeney et al., 2002). The second theoretical line proposes a functional shift, such that brain regions of the absent sense reorganize to process higher-order cognitive tasks, for example working memory tasks (Twomey et al., 2017). There is empirical support for both theoretical claims, and it has been suggested that they can coexist (Cardin et al., 2020). The present study was not designed to specifically answer the question of whether auditory cortex reorganizes to functionally preserve or shift functions. However, we did find changed functional connectivity between the superior temporal cortex and several different brain networks. This might indicate a functional shift where the superior temporal cortex can support several other networks and functions in the absence of auditory input. If on the other side, the auditory cortex reorganizes to preserve functions in a new sensory modality, we would expect to find group differences in connectivity between sensory cortices, such as the visual cortex and J. Andin and E. Holmer
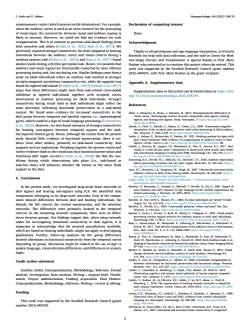
J.Andin and E Holmer 166 (2022)108139 Declaration of competing interes None. This is in co t to pr Acknowledgment orted s ectivity for deaf d to hea Thanks to all part ts and sign lar to Vic mory task ().and elp wit a collecti Bola et al (201 audi r who entru nding om 2015-00929),with Prof.Mary Rudner as the gant recipient. e th Appendix A.Supplementary data ArgU ha ight stem fro 8 References i,2013.Ne anguage pro ,A.,Flo ring A.,Kr reg th little of functio the is ev nly on th the Leppe Matsu Pike,B.,Zatorre,R l is it with m, nal shift ist (Card )but that the ditions during support in the data T 5.Conclusions is of th In the present study,we investigated lar cale brain netw orks in and sing ICA. We id f th .Dy er,P.C.,2006.Do deaf individualss better?Tren he laf D. e nd how?Nat.Rev. were primarily located the ing ne ackn edee tha the netw 1.L,2017.Ta s.Further.follow d ved alterations i ivity from the emporal c nces,and differences in sens Credit author statement B.,20 er,M.Woll,B 2013. Funding
Neuropsychologia 166 (2022) 108139 8 somatosensory cortex (which process tactile information). For example, when the auditory cortex is used as an extra resource for the processing of visual input, the connectivity between visual and auditory regions is likely to increase. However, we could not find any evidence for such reorganization. This is in contrast to previous task-based findings from both ourselves and others (Andin et al., 2021; Bola et al., 2017). We previously reported stronger connectivity for deaf compared to hearing individuals between the auditory cortex and visual cortices during a working memory task (Andin et al., 2021), and Bola et al. (2017) found similar results during a rhythm perception task. Hence, it is possible that auditory and visual regions are functionally connected by more efficient processing during task, but not during rest. Similar findings come from a study on blind individuals where an auditory task resulted in stronger occipito-temporal correlations compared to rest, while the opposite was found for sighted individuals (Pelland et al., 2017). Pelland et al. (2017) argue that these differences might stem from task-related cross-modal inhibition in sighted individuals together with occipital cortex involvement in auditory processing for blind individuals. Stronger connectivity during visual tasks in deaf individuals might reflect the same processes, indicating functional preservation in a task-based context. We found some evidence for increased connectivity in the deaf group between temporal and parietal regions, i.e., supramarginal gyrus, which could be a sign of visual language processing (Trettenbrein et al., 2021). However, we also found instances of stronger connectivity for hearing non-signers between temporal regions and the middle/superior frontal gyrus. Hence, although the results from the present study showed little evidence of functional preservation, there is evidence from other studies, primarily on task-based connectivity, that supports such an explanation. Weighing together the present results and earlier evidence leads to the conclusion that functional preservation and functional shift might co-exist (Cardin et al., 2020), but that the conditions during which observations take place (i.e., task-based or task-free data) will influence whether the former or the latter finds support in the data. 5. Conclusions In the present study, we investigated large-scale brain networks in deaf signers and hearing non-signers using ICA. We identified nine components belonging to six large-scale networks. Four of the components showed differences between deaf and hearing individuals; the default, the left control, the ventral somatomotor, and the attention networks. The differences were primarily located to the temporal cortices. In the remaining network components, there were no differences between groups. Our findings suggest that, when using network nodes for investigating functional connectivity in deaf signers, it is important to acknowledge that the network parcellations available, which are based on hearing individuals, might not apply to deaf signing populations. Further, follow-up analyses on the group differences showed alterations in functional connectivity from the temporal cortex depending on group. Alterations might be related to the use of sign vs spoken language, visual attention differences, and differences in sensory input. Credit author statement Josefine Andin: Conceptualization, Methodology, Software, Formal analysis, Investigation, Data curation, Writing – original draft, Visualization, Project administration, Funding acquisition. Emil Holmer: Conceptualization, Methodology, Software, Writing – review & editing. Funding This work was supported by the Swedish Research Council (grant number 2015–00929). Declaration of competing interest None. Acknowledgment Thanks to all participants and sign language interpreters, to Victoria Stenback ¨ for help with data collection, and the staff at Center for Medical Image Science and Visualization. A special thanks to Prof. Mary Rudner who entrusted us to continue this project when she retired. This work was supported by the Swedish Research Council (grant number 2015–00929), with Prof. Mary Rudner as the grant recipient. Appendix A. Supplementary data Supplementary data to this article can be found online at https://doi. org/10.1016/j.neuropsychologia.2021.108139. References Allen, J., Emmorey, K., Bruss, J., Damasio, H., 2013. Neuroanatomical differences in visual, motor, and language cortices between congenitally deaf signers, hearing signers, and hearing non-signers. Front. Neuroanat. 7 https://doi.org/10.3389/ fnana.2013.00026. Amedi, A., Floel, A., Knecht, S., Zohary, E., Cohen, L.G., 2004. Transcranial magnetic stimulation of the occipital pole interferes with verbal processing in blind subjects. Nat. Neurosci. 7, 1266–1270. https://doi.org/10.1038/nn1328. Andin, J., Holmer, E., Schonstr ¨ om, ¨ K., Rudner, M., 2021. Working memory for signs with poor visual resolution: fMRI evidence of reorganization of auditory cortex in deaf signers. Cerebr. Cortex 1–12. https://doi.org/10.1093/cercor/bhaa400, 00. Andoh, J., Ferreira, M., Leppert, I.R., Matsushita, R., Pike, B., Zatorre, R.J., 2017. How restful is it with all that noise? Comparison of Interleaved silent steady state (ISSS) and conventional imaging in resting-state fMRI. Neuroimage 147, 726–735. https:// doi.org/10.1016/J.NEUROIMAGE.2016.11.065. Armstrong, B.A., Neville, H.J., Hillyard, S.A., Mitchell, T.V., 2002. Auditory deprivation affects processing of motion, but not color. Cognit. Brain Res. 14, 422–434. https:// doi.org/10.1016/S0926-6410(02)00211-2. Auer, E.T., Bernstein, L.E., Sungkarat, W., Singh, M., 2007. Vibrotactile activation of the auditory cortices in deaf versus hearing adults. Neuroreport 18, 645–648. https:// doi.org/10.1097/WNR.0b013e3280d943b9. Bagga-Gupta, S., 2004. Literacy and Deaf Education - A Theoretical Analysis of the International and Swedish Literature. Bavelier, D., Brozinsky, C., Tomann, A., Mitchell, T., Neville, H., Liu, G., 2001. Impact of early deafness and early exposure to sign language on the cerebral organization for motion processing. J. Neurosci. 21, 8931–8942. https://doi.org/10.1523/ jneurosci.21-22-08931.2001. Bavelier, D., Dye, M.W.G., Hauser, P.C., 2006. Do deaf individuals see better? Trends Cognit. Sci. 10, 512–518. https://doi.org/10.1016/j.tics.2006.09.006. Bavelier, D., Neville, H.J., 2002. Cross-modal plasticity: where and how? Nat. Rev. Neurosci. 3, 443–452. https://doi.org/10.1038/nrn848. Benetti, S., Zonca, J., Ferrari, A., Rezk, M., Rabini, G., Collignon, O., 2021. Visual motion processing recruits regions selective for auditory motion in early deaf individuals. Neuroimage 230, 117816. https://doi.org/10.1016/j.neuroimage.2021.117816. Bola, L., Zimmermann, M., Mostowski, P., Jednorog, K., Marchewka, A., Rutkowski, P., Szwed, M., 2017. Task-specific reorganization of the auditory cortex in deaf humans. Proc. Natl. Acad. Sci. U. S. A. 114, E600–e609. https://doi.org/10.1073/ pnas.1609000114. Bonna, K., Finc, K., Zimmermann, M., Bola, L., Mostowski, P., Szul, M., Rutkowski, P., Duch, W., Marchewka, A., Jednorog, ´ K., Szwed, M., 2020. Early deafness leads to reshaping of functional connectivity beyond the auditory cortex. Brain Imaging Behav 87–100. https://doi.org/10.1007/s11682-020-00346-y. Bottari, D., Heimler, B., Caclin, A., Dalmolin, A., Giard, M.H., Pavani, F., 2014. Visual change detection recruits auditory cortices in early deafness. Neuroimage 94, 172–184. https://doi.org/10.1016/j.neuroimage.2014.02.031. Cardin, V., Grin, K., Vinogradova, V., Manini, B., 2020. Crossmodal reorganisation in deafness: mechanisms for functional preservation and functional change. Neurosci. Biobehav. Rev. 113, 227–237. https://doi.org/10.1016/j.neubiorev.2020.03.019. Cardin, V., Orfanidou, E., Ronnberg, ¨ J., Capek, C.M., Rudner, M., Woll, B., 2013. Dissociating cognitive and sensory neural plasticity in human superior temporal cortex. Nat. Commun. 4, 1473. https://doi.org/10.1038/ncomms2463. Cardin, V., Rudner, M., De Oliveira, R.F., Andin, J., Su, M.T., Beese, L., Woll, B., Ronnberg, ¨ J., 2018. The organization of working memory networks is shaped by early sensory experience. Cerebr. Cortex 28, 3540–3554. https://doi.org/10.1093/ cercor/bhx222. Corina, D.P., McBurney, S.L., Dodrill, C., Hinshaw, K., Brinkley, J., Ojemann, G., 1999. Functional roles of Broca’s area and SMG: evidence from cortical stimulation mapping in a deaf signer. Neuroimage 10, 570–581. https://doi.org/10.1006/ nimg.1999.0499. Dell Ducas, K., Senra Filho, A.C. da S., Silva, P.H.R., Secchinato, K.F., Leoni, R.F., Santos, A.C., 2021. Functional and structural brain connectivity in congenital J. Andin and E. Holmer
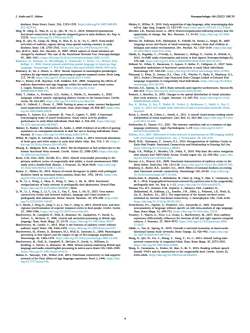
166(202)108139 261323-133.hp//n0.1070429 27 Me dM. M.W.C al Netw 179 tion in C n88 667- efault-n 201 of sigr chol.11 hi white .KE.200 2018.1 a 10. Strie M.R,Lash w..zi e Fisch.B. in,P.C.,P 3.1988-199 lin,V.2 is the f ory cortex witho e,P..D vid.A.,Williams
Neuropsychologia 166 (2022) 108139 9 deafness. Brain Struct. Funct. 226, 1323–1333. https://doi.org/10.1007/s00429- 021-02243-6. Ding, H., Ming, D., Wan, B., Li, Q., Qin, W., Yu, C., 2016. Enhanced spontaneous functional connectivity of the superior temporal gyrus in early deafness. Sci. Rep. 6, 23239. https://doi.org/10.1038/srep23239. Ding, H., Qin, W., Liang, M., Ming, D., Wan, B., Li, Q., Yu, C., 2015. Cross-modal activation of auditory regions during visuo-spatial working memory in early deafness. Brain 138, 2750–2765. https://doi.org/10.1093/brain/awv165. Dye, M.W.G., Baril, D.E., Bavelier, D., 2007. Which aspects of visual attention are changed by deafness? The case of the Attentional Network Test. Neuropsychologia 45, 1801–1811. https://doi.org/10.1016/j.neuropsychologia.2006.12.019. Emmorey, K., Damasio, H., McCullough, S., Grabowski, T., Ponto, L.L., Hichwa, R.D., Bellugi, U., 2002. Neural systems underlying spatial language in American Sign Language. Neuroimage 17, 812–824 https://doi.org/S1053811902911870 [pii]. Emmorey, K., Xu, J., Braun, A., 2011. Neural responses to meaningless pseudosigns: evidence for sign-based phonetic processing in superior temporal cortex. Brain Lang. 117, 34–38. https://doi.org/10.1016/j.bandl.2010.10.003. Fine, I., Finney, E.M., Boynton, G.M., Dobkins, K.R., 2005. Comparing the effects of auditory deprivation and sign language within the auditory and visual cortex. J. Cognit. Neurosci. 17, 1621–1637. https://doi.org/10.1162/ 089892905774597173. Finkl, T., Hahne, A., Friederici, A.D., Gerber, J., Mürbe, D., Anwander, A., 2020. Language without speech: segregating distinct circuits in the human brain. Cerebr. Cortex 30, 812–823. https://doi.org/10.1093/cercor/bhz128. Gaab, N., Gabrieli, J., Glover, G., 2008. Resting in peace or noise: scanner background noise suppresses default-mode network. Hum. Brain Mapp. 29, 858–867. https://doi. org/10.1002/HBM.20578. Gougoux, F., Zatorre, R.J., Lassonde, M., Voss, P., Lepore, F., 2005. A functional neuroimaging study of sound localization: visual cortex activity predicts performance in early-blind individuals. PLoS Biol. 3, 324–333. https://doi.org/ 10.1371/journal.pbio.0030027. Holmer, E., Rudner, M., Schonstr ¨ om, ¨ K., Andin, J., 2020. Evidence of an effect of gaming experience on visuospatial attention in deaf but not in hearing individuals. Front. Psychol. 11 https://doi.org/10.3389/fpsyg.2020.534741. Hribar, M., Suput, D., Carvalho, A.A., Battelino, S., Vovk, A., 2014. Structural alterations of brain grey and white matter in early deaf adults. Hear. Res. 318, 1–10. https:// doi.org/10.1016/j.heares.2014.09.008. Hwang, K., Hallquist, M.N., Luna, B., 2013. The development of hub architecture in the human functional brain network. Cerebr. Cortex 23, 2380–2393. https://doi.org/ 10.1093/cercor/bhs227. Karns, C.M., Dow, M.W., Neville, H.J., 2012. Altered cross-modal processing in the primary auditory cortex of congenitally deaf adults: a visual-somatosensory fMRI study with a double-flash illusion. J. Neurosci. 32, 9626–9638. https://doi.org/ 10.1523/JNEUROSCI.6488-11.2012. Kumar, U., Mishra, M., 2018. Pattern of neural divergence in adults with prelingual deafness: based on structural brain analysis. Brain Res. 1701, 58–63. https://doi. org/10.1016/j.brainres.2018.07.021. Li, W., Li, J., Wang, J., Zhou, P., Wang, Z., Xian, J., He, H., 2016. Functional reorganizations of brain network in prelingually deaf adolescents. Neural Plast. https://doi.org/10.1155/2016/9849087, 2016. Li, W., Li, J., Wang, Z., Li, Y., Liu, Z., Yan, F., Xian, J., He, H., 2015. Grey matter connectivity within and between auditory, language and visual systems in prelingually deaf adolescents. Restor. Neurol. Neurosci. 33, 279–290. https://doi. org/10.3233/RNN-140437. Li, Y., Booth, J., Peng, D., Zang, Y., Li, J., Yan, C., Ding, G., 2013. Altered intra- and interregional synchronization of superior temporal cortex in deaf people. Cerebr. Cortex 23, 1988–1996. https://doi.org/10.1093/cercor/bhs185. MacSweeney, M., Campbell, R., Woll, B., Brammer, M., Giampietro, V., David, A., Calvert, G., McGuire, P., 2006. Lexical and sentential processing in British sign language. Hum. Brain Mapp. 27, 63–76. https://doi.org/10.1002/hbm.20167. MacSweeney, M., Cardin, V., 2015. What is the function of auditory cortex without auditory input? Brain 138, 2468–2470. https://doi.org/10.1093/brain/awv197. MacSweeney, M., Waters, D., Brammer, M.J., Woll, B., Goswami, U., 2008. Phonological processing in deaf signers and the impact of age of first language acquisition. Neuroimage 40, 1369–1379. https://doi.org/10.1016/j.neuroimage.2007.12.047. MacSweeney, M., Woll, B., Campbell, R., McGuire, P., David, A., Williams, S., Suckling, J., Calvert, G., Brammer, M., 2002. Neural systems underlying British sign language and audio-visual English processing in native users. Brain 125, 1583–1593. https://doi.org/10.1093/brain/awf153. Malaia, E., Talavage, T.M., Wilbur, R.B., 2014. Functional connectivity in task-negative network of the Deaf: effects of sign language experience. PeerJ 2, e446. https://doi. org/10.7717/peerj.446. Malaia, E., Wilbur, R., 2010. Early acquisition of sign language: what neuroimaging data tell us. Sign Lang. Linguist 13, 183–199. https://doi.org/10.1075/sll.13.2.03mal. Merabet, L.B., Pascual-Leone, A., 2010. Neural reorganization following sensory loss: the opportunity of change. Nat. Rev. Neurosci. 11, 44–52. https://doi.org/10.1038/ nrn2758. Meristo, M., Falkman, K.W., Hjelmquist, E., Tedoldi, M., Surian, L., Siegal, M., 2007. Language access and theory of mind reasoning: evidence from deaf children in bilingual and oralist environments. Dev. Psychol. 43, 1156–1169. https://doi.org/ 10.1037/0012-1649.43.5.1156. Okada, K., Rogalsky, C., O’Grady, L., Hanaumi, L., Bellugi, U., Corina, D., Hickok, G., 2016. An fMRI study of perception and action in deaf signers. Neuropsychologia 82, 179–188. https://doi.org/10.1016/J.NEUROPSYCHOLOGIA.2016.01.015. Pelland, M., Orban, P., Dansereau, C., Lepore, F., Bellec, P., Collignon, O., 2017. Statedependent modulation of functional connectivity in early blind individuals. Neuroimage 147, 532–541. https://doi.org/10.1016/j.neuroimage.2016.12.053. P´enicaud, S., Klein, D., Zatorre, R.J., Chen, J.-K., Witcher, P., Hyde, K., Mayberry, R.I., 2012. Author’s Personal Copy Structural Brain Changes Linked to Delayed First Language Acquisition in Congenitally Deaf Individuals. https://doi.org/10.1016/j. neuroimage.2012.09.076. Petersen, S.E., Sporns, O., 2015. Brain networks and cognitive architectures. Neuron 88, 207–219. https://doi.org/10.1016/j.neuron.2015.09.027. Proksch, J., Bavelier, D., 2002. Changes in the spatial distribution of visual attention after early deafness. J. Cognit. Neurosci. 14, 687–701. https://doi.org/10.1162/ 08989290260138591. Ray, K., McKay, D., Fox, P., Riedel, M., Uecker, A., Beckmann, C., Smith, S., Fox, P., Laird, A., 2013. ICA model order selection of task co-activation networks. Front. Neurosci. Reich, L., Szwed, M., Cohen, L., Amedi, A., 2011. A ventral visual stream reading center independent of visual experience. Curr. Biol. 21, 363–368. https://doi.org/10.1016/ j.cub.2011.01.040. Schonstr ¨ om, ¨ K., Hauser, P.C., 2021. The sentence repetition task as a measure of sign language proficiency. Appl. Psycholinguist. 1–19 https://doi.org/10.1017/ S0142716421000436. Shibata, D.K., 2007. Differences in brain structure in deaf persons on MR imaging studied with voxel-based morphometry. Am. J. Neuroradiol. 28, 243–249. Shiell, M.M., Champoux, F., Zatorre, R.J., 2014. Reorganization of Auditory Cortex in Early-Deaf People: Functional Connectivity and Relationship to Hearing Aid Use. https://doi.org/10.1162/jocn_a_00683. Singh, A.K., Phillips, F., Merabet, L.B., Sinha, P., 2018. Why does the cortex reorganize after sensory loss? HHS Public Access. Trends Cognit. Sci. 22, 569–582. https://doi. org/10.1016/j.tics.2018.04.004. Stevens, A.A., Weaver, K.E., 2009. Functional characteristics of auditory cortex in the blind. Behav. Brain Res. 196, 134–138. https://doi.org/10.1016/j.bbr.2008.07.041. Stolzberg, D., Butler, B.E., Lomber, S.G., 2018. Effects of neonatal deafness on restingstate functional network connectivity. Neuroimage 165, 69–82. https://doi.org/ 10.1016/j.neuroimage.2017.10.002. Striem-Amit, E., Almeida, J., Belledonne, M., Chen, Q., Fang, Y., Han, Z., Caramazza, A., Bi, Y., 2016. Topographical functional connectivity patterns exist in the congenitally, prelingually deaf. Sci. Rep. 6, 1–13. https://doi.org/10.1038/srep29375. Thomas Yeo, B.T., Krienen, F.M., Sepulcre, J., Sabuncu, M.R., Lashkari, D., Hollinshead, M., Roffman, J.L., Smoller, J.W., Zollei, ¨ L., Polimeni, J.R., Fisch, B., Liu, H., Buckner, R.L., 2011. The organization of the human cerebral cortex estimated by intrinsic functional connectivity. J. Neurophysiol. 106, 1125–1165. https://doi.org/10.1152/jn.00338.2011. Trettenbrein, P.C., Papitto, G., Friederici, A.D., Zaccarella, E., 2021. Functional neuroanatomy of language without speech: an ALE meta-analysis of sign language. Hum. Brain Mapp. 42, 699–712. https://doi.org/10.1002/hbm.25254. Twomey, T., Waters, D., Price, C.J., Evans, S., MacSweeney, M., 2017. How auditory experience differentially influences the function of left and right superior temporal cortices. J. Neurosci. 37, 9564–9573. https://doi.org/10.1523/jneurosci.0846- 17.2017. Uddin, L., Yeo, B., Spreng, R., 2019. Towards a universal taxonomy of macro-scale functional human brain networks. Brain Topogr. 32, 926–942. https://doi.org/ 10.1007/S10548-019-00744-6. Wang, D., Qin, W., Liu, Y., Zhang, Y., Jiang, T., Yu, C., 2014. Altered resting-state network connectivity in congenital blind. Hum. Brain Mapp. 35, 2573–2581. https://doi.org/10.1002/hbm.22350. Wang, X., Caramazza, A., Peelen, M., Han, Z., Bi, Y., 2015. Reading without speech sounds: VWFA and its connectivity in the congenitally deaf. Cerebr. Cortex 25, 2416–2426. https://doi.org/10.1093/cercor/bhu044. J. Andin and E. Holmer
按次数下载不扣除下载券;
注册用户24小时内重复下载只扣除一次;
顺序:VIP每日次数-->可用次数-->下载券;
- 《认知心理学》课程教学资源(书籍文献)中国心理学家揭示面孔身份识别和表情识别的关联机制 Emerged human-like facial expression representation in a deep convolutional neural network.pdf
- 《认知心理学》课程教学资源(书籍文献)Science研究破解“脸盲”奥秘 A fast link between face perception and memory in the temporal pole.pdf
- 《认知心理学》课程教学资源(书籍文献)认知神经科学——关于心智的生物学(美)MICHAEL S.GAZZANIGA,Cognitive Neuroscience The Biology of the Mind(3rd Edition,彩色电子版).pdf
- 南方医科大学:《认知心理学》课程教学资源(课件讲稿)第一章 研究方法——认知神经科学(主讲:谭相良).pdf
- 《认知心理学》课程教学资源(书籍文献)The Student’s Guide to Cognitive Neuroscience,Fourth Edition,by Jamie Ward SY.pdf
- 《认知心理学》课程教学资源(书籍文献)Cognitive Neuroscience - The Biology of the Mind,Fourth Edition,Michael S. Gazzaniga, Richard B. Ivry, and George R. Mangun.pdf
- 《认知心理学》课程教学资源(书籍文献)认知科学与你的生活(原书第5版)美国名校学生最喜爱的心理学教材(美)凯瑟琳·加洛蒂(Kathleen M. Galotti)Cognitive Psychology - In and Out of the Laboratory,5th Edition.pdf
- 南方医科大学:《认知心理学》课程教学大纲 Cognitive psychology.pdf
- 《中国传统文化》课程教案讲义(打印版)第十六章 中外文化交流.pdf
- 《中国传统文化》课程教案讲义(打印版)第十一章 中国人生礼仪文化.pdf
- 《中国传统文化》课程教案讲义(打印版)第十章 中国饮食文化.pdf
- 《中国传统文化》课程教案讲义(打印版)第九章 中国服饰文化.pdf
- 《中国传统文化》课程教案讲义(打印版)第一章 文化简论.pdf
- 《中国传统文化》课程教学大纲(打印版)Introduction to Chinese Traditional Culture.pdf
- 《压力管理与心理健康》课程教学资源(教案讲义)第七章 消除压力,维护健康心理(打印版).pdf
- 《压力管理与心理健康》课程教学资源(教案讲义)第六章 压力自我调适的方法与技巧(打印版).pdf
- 《压力管理与心理健康》课程教学资源(教案讲义)第五章 认知重构与压力管理(打印版).pdf
- 《压力管理与心理健康》课程教学资源(教案讲义)第四章 人格特质与心理压力(打印版).pdf
- 《压力管理与心理健康》课程教学资源(教案讲义)第三章 大学生心理压力及应对(打印版).pdf
- 《压力管理与心理健康》课程教学资源(教案讲义)第二章 心理压力概述(打印版).pdf
- 《认知心理学》课程教学资源(书籍文献)反射性注意的返回抑制起始时间可显著预测认知老化 Onset Time of Inhibition of Return Is a Promising Index for Assessing Cognitive Functions in Older Adults.pdf
- 《认知心理学》课程教学资源(书籍文献)注意缺陷多动障碍的视觉注意网络研究新发现 Neural Correlates of the Dual-Pathway Model for ADHD in Adolescents.pdf
- 《认知心理学》课程教学资源(书籍文献)神经与精神疾病(精神疾病的愉快体验加工和社会认知).pdf
- 南方医科大学:《认知心理学》课程教学资源(课件讲稿)第一章 认知心理——研究方法.pdf
- 南方医科大学:《认知心理学》课程教学资源(课件讲稿)第七章 记忆的认知神经机制.pdf
- 南方医科大学:《认知心理学》课程教学资源(课件讲稿)第三章 大脑半球的偏侧化(主讲:张瑞彬).pdf
- 南方医科大学:《认知心理学》课程教学资源(课件讲稿)第九章 社会认知的神经机制.pdf
- 南方医科大学:《认知心理学》课程教学资源(课件讲稿)第五章 物体识别的认知神经机制.pdf
- 南方医科大学:《认知心理学》课程教学资源(课件讲稿)第八章 情绪的认知神经机制.pdf
- 南方医科大学:《认知心理学》课程教学资源(课件讲稿)第六章 注意的认知神经机制.pdf
- 南方医科大学:《认知心理学》课程教学资源(课件讲稿)第十章 决策的认知神经机制.pdf
- 南方医科大学:《认知心理学》课程教学资源(课件讲稿)第四章 感知觉的认知神经机制——以观觉为例.pdf
- 南方医科大学:《认知心理学》课程教学资源(课件讲稿)记忆的认知神经机制-课件-王优.pdf
- 山东第一医科大学(泰山医学院):《大学生心理健康教育》课程教学大纲 Mental Health Education of College Student.doc
- 山东第一医科大学(泰山医学院):《大学生心理健康教育》课程教学资源(授课教案)第1章 绪论(负责人:冯宪萍).doc
- 山东第一医科大学(泰山医学院):《大学生心理健康教育》课程教学资源(授课教案)第2章 新生与环境适应.doc
- 山东第一医科大学(泰山医学院):《大学生心理健康教育》课程教学资源(授课教案)第3章 自我意识与大学生心理健康.doc
- 山东第一医科大学(泰山医学院):《大学生心理健康教育》课程教学资源(授课教案)第4章 情绪与大学生心理健康.doc
- 山东第一医科大学(泰山医学院):《大学生心理健康教育》课程教学资源(授课教案)第5章 人格与大学生心理健康.doc
- 山东第一医科大学(泰山医学院):《大学生心理健康教育》课程教学资源(授课教案)第6章 人际交往与大学生心理健康.doc