《生物化学》课程教学资源(文献资料)聚焦肠道菌群与免疫代谢的互作(The Gut Microbiota at the Service of Immunometabolism)

CellPress Cell Metabolism Perspective The Gut Microbiota at the Service of Immunometabolism aMhagecaadiaosotaei 3Sorbonne Universite,INSERM,Centre de Recherche Saint-Antoine,CRSA,AP-HP,Saint Antoine Hospital,Gastroenterology Department, 75012 Paris,France SUMMARY The gut microbiota is implicated in immune system functions.Regulation of the metabolic processes occur- ring in immune cells is crucial for the maintenance of homeostasis and immunopathogenesis.Emerging data demonstrate that the gut microbiota is an actor in immunometabolism,notably through the effect of metab- olites such as short-chain fatty acids,bile acids,and tryptophan metabolites.In this Perspective,we discuss the impact of the gut microbiota on the intracellular metabolism of the different subtypes of immune cells, hi cell Besides the effectson heath,we discus the potentiaons nflammatory bowel diseases INTRODUCTION molecules,either produced or transformed by microorgan- isms.are maior actors in the dialog with immune cells Metabolism involves cellular mechanisms to sustain life during (Backhed et al.,2004;Cavallari et al.,2020;Lavelle and Sokol, physiological or pathological processes.More generally,it is 2020).Given the key role of the gut microbiome in physiolog- about eneray:the utilization of metabolic substrates.notably ical processes,any alteration in its composition or function glucose,fatty acids(FAs),and amino acids(AAs);and the bal could induce or participate in a disease(Pigneur and Sokol. ance between catabolism and anabolism that maintain cellular 2016).The alobal role of the aut microbiota in immunity has homeostasis.Metabolism is impacted by lifestyles and dietary been extensively reviewed (Honda and Littman,2016:Rooks habits,as illustrated by the increased rate of infection in and Garrett 2016)H clly discuss malnourished populations (Blanton et al.2016:Hashimoto of tha et al..2012)and the metabolic syndrome-related disease and pre verfed the ntial of immune cells,in 1n2002,im ations living in de elopedco with the glycolysis ENERGY ARCHITECTURE TO of immune c on met tabolism and,conversely,the meta Immune system development/activation typically involves needs of immune cells during homeostasis and pathological changes in the expression of large numbers of genes and results settings. in the acquisition of new functions,such as high production of The microbiome is a maior contributor to health.contrib cytokines.lipid mediators.and tissue-remodeling enzymes uting to several development processes,homeostatic states, and the ability to migrate through tissues and/or undergo cellular and responses to pathogenic situations.Although the human division.Immune cells use the same pathways as other cell types microbiome is composed of several microbiotas colonizing eray and ensure their effective functionina the different niches (e.g.,lung,skin,mouth,and vagina),the main metabolic pathways involved in immunometabolism are glycolysis,the tricarboxylic acid(TCA)cycle,the pentose phos- ate athw /(PPP).FA oxi (FAO),FA 201)and15.0m(arin 100 trillion mic synthesis,and th s im- 91 (Pasolli et al,2019) cting the e mete olism of The gut micr ia fu -chain protists (lliev a nardi,2017:Ri tryptophan etabolism,lipid meta We presen ill discuss s and refer the with its reader to recent extensive reviews on this topic for more details host.The gut microbiome plays a role in the modulation of (Bantug et al,2018;Goodpaster and Sparks,2017;O'Neill both metabolism and immunity.Indeed,microbiome-derived etal.2016). 514 Cell Metabolism 32,October 6,20202020 Elsevier Inc ®
Perspective The Gut Microbiota at the Service of Immunometabolism Chloe´ Michaudel1,2 and Harry Sokol1,2,3, * 1INRA, UMR1319 Micalis and AgroParisTech, Jouy en Josas, France 2Paris Center for Microbiome Medicine (PaCeMM) FHU, Paris, France 3Sorbonne Universite´ , INSERM, Centre de Recherche Saint-Antoine, CRSA, AP-HP, Saint Antoine Hospital, Gastroenterology Department, 75012 Paris, France *Correspondence: harry.sokol@aphp.fr https://doi.org/10.1016/j.cmet.2020.09.004 SUMMARY The gut microbiota is implicated in immune system functions. Regulation of the metabolic processes occurring in immune cells is crucial for the maintenance of homeostasis and immunopathogenesis. Emerging data demonstrate that the gut microbiota is an actor in immunometabolism, notably through the effect of metabolites such as short-chain fatty acids, bile acids, and tryptophan metabolites. In this Perspective, we discuss the impact of the gut microbiota on the intracellular metabolism of the different subtypes of immune cells, including intestinal epithelial cells. Besides the effects on health, we discuss the potential consequences in infection context and inflammatory bowel diseases. INTRODUCTION Metabolism involves cellular mechanisms to sustain life during physiological or pathological processes. More generally, it is about energy; the utilization of metabolic substrates, notably glucose, fatty acids (FAs), and amino acids (AAs); and the balance between catabolism and anabolism that maintain cellular homeostasis. Metabolism is impacted by lifestyles and dietary habits, as illustrated by the increased rate of infection in malnourished populations (Blanton et al., 2016; Hashimoto et al., 2012) and the metabolic syndrome-related disease outbreak in overfed populations living in developed countries. In 2002, immunometabolism, a new branch of metabolism, was brought to light with the discovery of the link between CD28 activation and glycolysis in T cells (Frauwirth et al., 2002, p. 2). This field notably aims to understand the impact of immune cells on metabolism and, conversely, the metabolic needs of immune cells during homeostasis and pathological settings. The microbiome is a major contributor to health, contributing to several development processes, homeostatic states, and responses to pathogenic situations. Although the human microbiome is composed of several microbiotas colonizing different niches (e.g., lung, skin, mouth, and vagina), the most studied is that in the gastrointestinal tract. It is composed of diverse microbial communities, approximately 100 trillion microorganisms (Sarin et al., 2019; Sender et al., 2016) and 150,000 microbial genomes (Pasolli et al., 2019). The gut microbiome is composed of bacteria, fungi, viruses, and protists (Iliev and Leonardi, 2017; Richard and Sokol, 2019; Shkoporov and Hill, 2019), and following millions of years of concomitant evolution, it is in symbiosis with its host. The gut microbiome plays a role in the modulation of both metabolism and immunity. Indeed, microbiome-derived molecules, either produced or transformed by microorganisms, are major actors in the dialog with immune cells (Backhed et al., 2004 € ; Cavallari et al., 2020; Lavelle and Sokol, 2020). Given the key role of the gut microbiome in physiological processes, any alteration in its composition or function could induce or participate in a disease (Pigneur and Sokol, 2016). The global role of the gut microbiota in immunity has been extensively reviewed (Honda and Littman, 2016; Rooks and Garrett, 2016). Here, we specifically discuss the effects of the gut microbiota on immunometabolism, and more precisely, on the intracellular metabolism of immune cells, in health and the potential consequences in diseases. IMMUNOMETABOLISM: ENERGY ARCHITECTURE TO PROMOTE IMMUNITY Immune system development/activation typically involves changes in the expression of large numbers of genes and results in the acquisition of new functions, such as high production of cytokines, lipid mediators, and tissue-remodeling enzymes, and the ability to migrate through tissues and/or undergo cellular division. Immune cells use the same pathways as other cell types to generate energy and ensure their effective functioning. The main metabolic pathways involved in immunometabolism are glycolysis, the tricarboxylic acid (TCA) cycle, the pentose phosphate pathway (PPP), FA oxidation (FAO), FA synthesis, and AA metabolism. Among the microbiome metabolism pathways impacting the metabolism of the immune cells, we will notably discuss short-chain fatty acid (SCFA) production, tryptophan metabolism, lipid metabolism, and bile acid (BA) transformation. We present here the main actors we will discuss and refer the reader to recent extensive reviews on this topic for more details (Bantug et al., 2018; Goodpaster and Sparks, 2017; O’Neill et al., 2016). ll 514 Cell Metabolism 32, October 6, 2020 ª 2020 Elsevier Inc

Cell Metabolism CelPress Perspective which is particularly relevant for lism pathways in specific immune cell types (Figure 1). inal epith ant actor in hos in nmune resp t is estimated t approx energy m the consumption of hNADH and FADH molecules he gut mic obiota.Early in life,before adaptive immu d by oxidative phosphorylation (OXPHOS)in mito- FA synthesis is required for the biosynthe sis of the cell in an IL-3-and IL-22-depend nembrane acti targe of signaling 22 production leads an abn mal lipid metabolism tion.It leads to the pro ugh the TCA cycle nd oxPHOS o rol is le,B-o and pyruvate dehy several biomole d BA 11). agy is ind rget d thr ough the oxidation of cholesterol.These mo les nn is d th are a goode they are synth but lonocytes )the the bile duct and re enzymes and damp 20- autopnagy h。 atic produce m us and antimicrobial peptides to maintain a are humar IECs (M et a 201) GR5).far r(FXR).and esides their b ing blocks le for se in nalina nat ays and me for the onin (5-HD which ha 0).Glutamine asp artate are see b feed the TCA cycle to producee or be a substrate fo -formin gbacteria metab mecha synthesis.Meta ofother AAs,such as arginine trypto date 2019:M For nin prod ion.can be achie d by SCFAs(b e mo ro ial hi d the 2015 ven if further in ns are needed,thes eads to the p ng the (AhR)agonists that exhibit immunomodulatory eff ng the production of serotonin from a therapeutic from en perspective n the er the influ blish adrectimm nomodulatory factor,with seven re crophages and non-immu Cell Metabolism 32.October 6,2020 515
Glycolysis is a relatively inefficient way to generate energy, as the breakdown of one unit of glucose produces only two ATP molecules (Lunt and Vander Heiden, 2011). However, it is a source of intermediate molecules for other pathways, including the PPP, AA, and FA metabolism pathways, and it can be swiftly activated, which is particularly relevant for proliferating cells such as T cells. The TCA cycle (or Krebs or citric acid cycle), which takes place in mitochondria in eukaryotes, is a crucial engine in energy generation. Its primary substrate is acetyl-CoA produced either from pyruvate by oxidative decarboxylation at the end of glycolysis or from FAO. It is estimated that the TCA cycle produces approximately 30 molecules of ATP from one molecule of glucose, including the consumption of the NADH and FADH2 molecules produced by oxidative phosphorylation (OXPHOS) in mitochondria. FA synthesis is required for the biosynthesis of the cell membrane, energy storage, and the generation of signaling molecules. This pathway is tightly dependent on mTOR (mammalian target of rapamycin) signaling and principally uses acetyl-CoA and other molecules provided by glycolysis, the TCA cycle, and the PPP. Beta-oxidation is the main metabolic pathway for FA degradation. It leads to the production of acetyl-CoA, NADH, and FADH2 and then to a high amount of energy through the TCA cycle and OXPHOS. Cholesterol is an essential precursor of several biomolecules, including steroid hormones, vitamin D, oxysterols, and BAs. BAs are produced through the oxidation of cholesterol. These molecules are a good example of co-metabolism, as they are synthesized as primary and conjugated BAs by the liver (Fiorucci et al., 2018); they reach the intestine through the bile duct and are converted by gut microbiota enzymes into unconjugated secondary BAs. Most of the BAs are reabsorbed in the terminal ileum and go back to the liver, completing their entero-hepatic cycle. Beyond their role in lipid digestion, BAs are signaling molecules impacting many immune cell types through several membranes and nuclear receptors, such as G protein-coupled BA receptor 5 (TGR5), farnesoid X receptor (FXR), and vitamin D receptor (VDR). Besides their building blocks role for proteins, some AAs are also precursors of bioactive molecules that contribute to the maintenance of signaling pathways and metabolism (Liu et al., 2020). Glutamine and aspartate are involved in nucleotide synthesis (Cory and Cory, 2006; Gots, 1971). Glutamine can also feed the TCA cycle to produce energy or be a substrate for FA synthesis. Metabolites of other AAs, such as arginine and tryptophan, are involved in cell proliferation and growth processes (Badawy, 2019; Milner, 1985). For example, tryptophan can be metabolized into a myriad of active molecules through three major pathways: the kynurenine pathway, the serotonin pathway, and the indole pathway. While the first two pathways occur in mammalian cells, the last pathway takes place in the gut microbiota and leads to the production of aryl hydrocarbon receptor (AhR) agonists that exhibit immunomodulatory effects (Agus et al., 2018). The production of serotonin from enterochromaffin cells in the gut is under the influence of the microbiome. It is well established as a direct immunomodulatory factor, with seven receptor isoforms expressed on immune and non-immune cell types (Shajib and Khan, 2015). KEY ROLES OF THE MICROBIOTA IN IMMUNE CELL METABOLISM Several recent studies highlighted newly discovered mechanisms by which the gut microbiota manipulates immunometabolism pathways in specific immune cell types (Figure 1). Epithelial Cells The gastrointestinal epithelium is a highly relevant actor in hostmicrobiome interactions; it is one of the first players in the immune response, and intestinal epithelial cells (IECs) are now considered immune cells (Allaire et al., 2018). The energy metabolism of IECs, particularly in the colon, is largely dependent on the gut microbiota. Early in life, before adaptive immune system maturation, unidentified microbiota-derived molecules activate intraepithelial lymphocytes (IELs) and ILC3 through STAT3 phosphorylation in an IL-23- and IL-22-dependent manner. In the absence of adaptive immunity, the IL-23-ILC3-IL-22-IEC circuit allows control of the gut microbiota, but the overactivated IL- 22 production leads to an abnormal lipid metabolism with reduced expression of key lipid transporters (e.g., CD36, Fabp1/2), and reduction of triglycerides and free FA in serum (Mao et al., 2018). In germ-free mice, colonocytes exhibit an energy-deprived state with decreased activity of enzymes of the TCA cycle, b-oxidation, and pyruvate dehydrogenase complex (Donohoe et al., 2011). Autophagy is induced by the energetic stress to maintain homeostasis in colonocytes. The SCFA butyrate produced by the gut microbiome in the colon is indeed the only source of carbon for colonocytes. After being transformed into butyryl-CoA, it diffuses passively into the mitochondria, undergoes b-oxidation, and feeds the TCA cycle and OXPHOS to produce energy and dampen autophagy activation (Donohoe et al., 2011). IECs are massively exposed to gut microbes and produce mucus and antimicrobial peptides to maintain a safety distance. Butyrate also promotes intestinal homeostasis by downregulating IDO1 expression and the kynurenine pathway in human IECs (Martin-Gallausiaux et al., 2018). The mechanisms involve a reduction in signal transducer and activator of transcription (STAT) 1 expression and HDAC (histone deacetylase) inhibition. Among the different IEC types, enterochromaffin (EC) cells are responsible for the production of serotonin (5-HT), which has major effects on immune cells (see below). Serotonin production in the colon is largely modulated by the gut microbiota and particularly spore-forming bacteria metabolites. The mechanisms are not fully elucidated, but it has been shown that upregulation of TpH1 expression, the rate-limiting enzyme in serotonin production, can be achieved by SCFAs (butyrate and propionate) and some secondary BAs, such as deoxycholate produced by microbial biotransformation of cholate (Yano et al., 2015). Even if further investigations are needed, these data suggest that modulating the gut microbiota composition or directly administrating microbial metabolites could allow manipulating the production of serotonin from a therapeutic perspective. Macrophages Macrophages are in the first line during the immune response but also sense and respond to the microbiota to control it without ll Cell Metabolism 32, October 6, 2020 515 Perspective
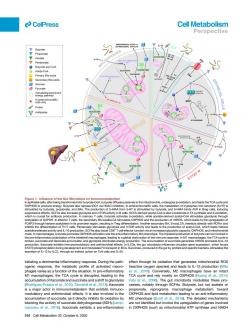
CellPress Cell Metabolism Perspective 2学 cetyt-CoA Protist ATP ot the Gut micr TA initiating a detrimental inflammatory response.During the path effect through its oxidation that generates mitochondrial ROS 】macf0 spec eaas to IL-1B production s.the TCA cycle is disrupted,leading to the TCA cycle and mostly on OXPHOS (Huang 2010T 201 taconate is a major actor in immunome bo ism that exhibits ir muno- reprograms macrophage vard n of suc ate as it di btsoxid M2 n (Scott et al 20 e(SDH)(Lamp are not ic d but involve the ropoulou et a 5 516 Cell Metabolism 2,October6,020
initiating a detrimental inflammatory response. During the pathogenic response, the metabolic profile of activated macrophages varies as a function of the situation. In pro-inflammatory M1 macrophages, the TCA cycle is disrupted, leading to the accumulation of itaconate and succinate and a shift to glycolysis (Rodrı´guez-Prados et al., 2010; Tannahill et al., 2013). Itaconate is a major actor in immunometabolism that exhibits immunomodulatory and antimicrobial effects. It is also involved in the accumulation of succinate, as it directly inhibits its oxidation by blocking the activity of succinate dehydrogenase (SDH) (Lampropoulou et al., 2016). Succinate exhibits a pro-inflammatory effect through its oxidation that generates mitochondrial ROS (reactive oxygen species) and leads to IL-1b production (Mills et al., 2016). Conversely, M2 macrophages have an intact TCA cycle and rely mostly on OXPHOS (Huang et al., 2014; Vats et al., 2006). The gut microbiota modulates these processes, notably through SCFAs. Butyrate, but not acetate or propionate, reprograms macrophage metabolism toward OXPHOS and lipid metabolism leading to an anti-inflammatory M2 phenotype (Scott et al., 2018). The detailed mechanisms are not identified but involve the upregulation of genes involved in OXPHOS (such as mitochondrial ATP synthase and NADH Figure 1. Influence of the Gut Microbiota on Immunometabolism In epithelial cells, after being transformed into butyrate-CoA, butyrate diffuses passively in the mitochondria, undergoes b-oxidation, and feeds the TCA cycle and OXPHOS to produce energy. Butyrate also repress IDO1 via HDAC inhibition. In enterochromaffin cells, the metabolism of tryptophan into serotonin (5-HT) is stimulated by butyrate, propionate, and BAs. The production of 5-HIAA from 5-HT is stimulated by butyrate, and 5-HIAA binds AhR in Breg cells, inducing suppressive effects. SCFAs also increase glycolysis and mTOR activity in B cells. SCFA-derived acetyl-CoA is also a substrate in FA synthesis and b-oxidation, which is crucial for antibody production. In memory T cells, butyrate activates b-oxidation, while acetate-derived acetyl-CoA stimulates glycolysis through acetylation of GAPDH. In effector T cells, the secondary BA isoalloLCA stimulates OXPHOS and the production of mtROS, which leads to the upregulation of FOXP3 through histone acetylation in its promoter region, resulting in Treg differentiation. Another secondary BA, 3-oxoLCA, interacts directly with RORgt and inhibits the differentiation of Th17 cells. Pentanoate stimulates glycolysis and mTOR activity and leads to the production of acetyl-CoA, which feeds histone acetyltransferase activity and IL-10 production. SCFAs also boost CD8+ T cell effector function via an increased glycolytic capacity, OXPHOS, and mitochondrial mass. In macrophages, butyrate promotes OXPHOS activation and the anti-inflammatory M2 phenotype. The impaired production of butyrate can be involved in the pro-inflammatory polarization of the intestinal macrophages, leading to a global dysfunction of the immune response. In M1 macrophages, the TCA cycle is broken, succinate and itaconate accumulate, and glycolysis dominates energy production. The accumulation of succinate generates mtROS and leads to IL-1b production. Itaconate exhibits immunomodulatory and antimicrobial effects. In ILC3s, the gut microbiota influences circadian gene expression, which favors STAT3 phosphorylation during development and modulates FA transport in IECs. Succinate, produced in the gut by protists and specific bacteria, stimulates the secretion of IL-13 by ILC2, through an indirect action on Tuft cells and IL-25. ll 516 Cell Metabolism 32, October 6, 2020 Perspective

Cell Metabolism CelPress Perspective dehyrdirogenase butyrate ind d by antibiotics promotes the pro-inflammato T cell acti mainly through RO production (Sena ta TCA intake enzymes. e0Saleectron red activation.ROS act in syn with calciu nflux to elicit IL-2 te Lymphoid Cell types of innate lymphoid cells (ILCs)charac Microbiota-derived SCFAs boost CDa cell effector fun ized by the exp ssion of specr me ane sm (Tromp et ation.ILCs change theirnerg netabolism profo to fit that .20 ell as the mecha d and amino acid n sm.and ILC3 SCFA can diffus glycolysis bl. the cyt and s ects of antibiotics on the transcriptomic C1 As. ularly butyrate,boost th e of ILC t in the e IL-17 and IL-22,and are eg atabolisn tan ine utilization. se and 1 to me (Bachem et) ntenance f the m de in stre amoun of acetate is (ari hydr arbon recepto rnuclear tran like Liah tate uptake memory CD8 cells expands the acetyl-CoA k cycles are key factors in this process,but the gut micro citrate lya tal 2016).also has some impact (Godinho-Silva et a 019 enzyme in glycolysis. The p call ca city CD of systemic lipid m etabolis et al 20170 bed with host tate,they are likely trig ut mi rate mo nct at le g te pro and asthma.The m m termi n mitoc ROS(mROS)prod on and glycolysis b et al.. me ems involve the inhibiti of HDAC onal has hoo ntly sho rectly tue TCA h pent wn as valerate).a subo nt m cific ba eria stimulates the secretion of ll-13 C2.thr anti-inflam matory cytokine L-10 by and IL-5( retaonLc2 for and en cing glycolysi remains to be explored. arding the activation of mTOR by SCFAs T cell metabolic plasticity is necessary to fit the which are inhibitor and activator of AMP-activa dprote une e vely pa and REDOX etal,2019).Effecto (K m et al.,2016;Lu uet a :Zhou eta 2018).The seconc I Tunctions P300/CBP (E1A glycolysis in effectorTcells and by FAOand OXPHOS inme orotein P300/CREB-b ding protein) etylationof t of mTOR.ading to more atin of the Cell Metabolism 32.October 6,2020 517
dehydrogenase) and lipid metabolism (such as lipoprotein lipase) pathways. As an illustration, the impaired production of butyrate induced by antibiotics promotes the pro-inflammatory polarization of the intestinal macrophages, leading to a global dysfunction of the immune response (Scott et al., 2018). This might play a role in the association between antibiotics intake and the emergence of inflammatory and metabolic diseases (Cox et al., 2014; Hviid et al., 2011). Innate Lymphoid Cells There are different types of innate lymphoid cells (ILCs) characterized by the expression of specific membrane markers, transcription factors, and cytokine signatures. During their activation, ILCs change their energy metabolism profoundly to fit their new functions (Rolot and O’Sullivan, 2020). Transcriptomic analysis suggests that ILC1s use mTOR signaling, ILC2s depend on sphingolipid and amino acid metabolism, and ILC3s rely on glycolysis (Gury-BenAri et al., 2016). The gut microbiota profoundly impacts ILC function as demonstrated by the dramatic effects of antibiotics on the transcriptomic program of ILC1s, ILC2s, and ILC3s (Gury-BenAri et al., 2016). ILC3 is the main type of ILC present in the gastrointestinal tract. These cells express RORgt, can produce IL-17 and IL-22, and are crucial regulators of inflammation, infection, microbiota composition, and metabolism (Klose and Artis, 2016). ILC3 functions, such as maintenance of the intestinal epithelium defense, depend on circadian signals mediated by the circadian regulator ARNTL (aryl hydrocarbon receptor nuclear translocator like). Lightdark cycles are key factors in this process, but the gut microbiota, which is known to be an actor in diurnal rhythmicity (Thaiss et al., 2016), also has some impact (Godinho-Silva et al., 2019). This signaling circuit connecting the gut microbiota, ILC3, and the intestinal epithelial clock is also involved in the regulation of the local and systemic lipid metabolism (Wang et al., 2017). Gut microbiota-derived butyrate modulates ILC2 functions, inhibiting their uncontrolled activation and, consequently, their negative role in lung inflammation and asthma. The mechanism is not determined. Yet the involvement of intracellular metabolism is supported by the induction of changes in mitochondrial ROS (mROS) production and glycolysis by butyrate (Lewis et al., 2019). Moreover, the preferential use of FAs over glucose by ILC2 to maintain their function in infection or nutritional stress suggests that butyrate might directly fuel the TCA (Wilhelm et al., 2016). Succinate, produced in the gut by protists and specific bacteria, stimulates the secretion of IL-13 by ILC2, through an indirect action on Tuft cells and IL-25 (Schneider et al., 2018). The role of succinate of other origin and its direct impact on ILC2 remains to be explored. T Cells T cell metabolic plasticity is necessary to fit the permanently dynamic immune environment. The gut microbiota actively participates in this programming via ROS, SCFA, and BA production and REDOX signaling modification (Skelly et al., 2019). Effector and memory T cells have very different functions and needs and thus exhibit different metabolism. It is dominated by aerobic glycolysis in effector T cells and by FAO and OXPHOS in memory T cells. Mitochondrial dynamics are evidence of these differences, with fused mitochondrial networks in memory T cells and punctate mitochondria in effector T cells (Buck et al., 2016). In addition, mitochondria are a critical component of T cell activation, mainly through ROS production (Sena et al., 2013). T cell stimulation via CD3 induces calcium influx that stimulates the function of pyruvate dehydrogenase and TCA enzymes. TCA cycling activates the mitochondrial electron transport chain and leads to the production of ROS, which are required for T cell activation. ROS act in synergy with calcium influx to elicit IL-2 expression, likely in an NF-kB- and AP-1- dependent manner (Kaminski et al., 2010). Microbiota-derived SCFAs boost CD8+ T cell effector functions by modifying their cellular metabolism (Trompette et al., 2018). SCFAs produced by the metabolism of dietary fibers by the gut microbiota stimulate OXPHOS and mitochondrial mass in CD8+ T cells as well as their glycolytic capacity. The mechanisms are not yet fully understood, but a part of these changes depend on GPR41 activation. Besides, SCFAs can diffuse into the cytoplasm and serve as a substrate for FAO, leading to the production of acetyl-CoA that fuel TCA and then OXPHOS. In activated CD8+ T cells, SCFAs, particularly butyrate, boost the uptake and oxidation of FAs, leading to a disconnection of the TCA cycle from glycolytic input and favoring OXPHOS through FA catabolism and glutamine utilization. This butyrate-induced cellular metabolism adaptation is required for the differentiation to memory T cells (Bachem et al., 2019). In stress situations, a massive amount of acetate is released into the extracellular space via hydrolysis from acetyl-CoA. Acetate uptake by memory CD8+ T cells expands the acetyl-CoA pool though TCA cycle and ATP citrate lyase activity and triggers the acetylation of GAPDH (glyceraldehyde 3-phosphate dehydrogenase), a key enzyme in glycolysis. The prompt stimulation of glycolysis allows the rapid recall capacity of CD8+ memory T cells (Balmer et al., 2016). Although these phenomena were described with host cell-derived acetate, they are likely triggered, at least in the gut, by the massive amount of acetate produced by the gut microbiota. SCFAs also exhibit significant effects on CD4+ T cells, notably regarding the generation of T helper (Th) 17, Th1 (Park et al., 2015), and regulatory T cells (Furusawa et al., 2013; Smith et al., 2013). The mechanisms involve the inhibition of HDACs and regulation of the mTOR pathway (a master regulator of cell growth and metabolism). This link has been recently shown with pentanoate (also known as valerate), a subdominant microbiota-produced SCFA that can stimulate the production of the anti-inflammatory cytokine IL-10 by providing additional acetylCoA for histone acetyltransferases and enhancing glycolysis and mTOR activity (Luu et al., 2019). Two mechanisms have been suggested regarding the activation of mTOR by SCFAs (Figure 2). Through their action on energy production pathways, SCFAs induce the production of ATP and the depletion of AMP, which are inhibitor and activator of AMP-activated protein kinase (AMPK), respectively. Consequently, the inhibitor activity of AMPK on mTOR is repressed, thus leading to mTOR activation (Kim et al., 2016; Luu et al., 2019; Zhou et al., 2018). The second potential mechanism involves the HDAC inhibition activity of SCFAs. SCFAs, in association with P300/CBP (E1A binding protein p300/CREB-binding protein), promote acetylation of the ribosomal protein S6 kinase beta-1 (S6K1), which is a downstream target of mTOR, leading to more robust activation of the ll Cell Metabolism 32, October 6, 2020 517 Perspective

CellPress Cell Metabolism Perspective AMP↓+ NUTRIENT DEPRIVATION AMPK PRODUCTION ce the ATP个 are in mTOR BA R is rep d,thus leading CFAS.in ⊙ P300/CBP of the AUTOPHAGY ULK1 B E act P 4EBP 点 (P PHOSPHORYLATION pathway(Park et al.2015).Another laver of complexity has beer of IL-10-producing Breas (regulatory B cells).In Breas,Rosse and col eagues recenty sho ed that butyrate could divert tryp at al 2020) ction of 5-hydroxyindole-aceiccd As oha entia mpact onT ta,20 0).Surprisingly.5-HIAA was shown to activate AhF Th17cells by directly interacting with the transcription facto plementation in a rheumatoid arthritis model in vivo. yt (H Co rsely,ano den CONSEQUENCES FOR DISEASE PATHOGENESIS mechanism involves the stimulation of OXPHOS and the increa mmun the ene et al.. 2019).In the colon specifically matory and infect diseases is much higher,and th whole recepto eg to reguat nanceof ers and in the(.) As seen above B Cells can have positive or negative effects on these pathological ntiation into plasma cels and the events(Figure 3). Infections o fuel the Gellareneryengneatdtierer se pro drial TC eto intestinal pathogens.Their cation within the intes. production of ATP SCFAs alsc which are ependent on the gu A is also a substrate in FA(parti acid)s 7).Upon infectior tad ce and e in ass sed on genetic are de dent on mTORn micr bial ghlight a co h cell functions.Th n in creprogramming ofs to boost the olic changes the surface of innate imr e cells uch as r nfection.In the early steps of inf on with the n pathoger in tolerance to and import activated ction of immunogl 518 Cell Metabolism 2,October6,020
pathway (Park et al., 2015). Another layer of complexity has been indicated recently by showing that the effects of SCFAs on T cell metabolism are dependent on the inflammatory context (Trapecar et al., 2020). BAs also have an essential impact on T cells. A derivative of lithocholic acid (LCA), 3-oxoLCA, inhibits the differentiation of Th17 cells by directly interacting with the transcription factor RORgt (Hang et al., 2019). Conversely, another derivative of LCA, isoalloLCA, promotes the differentiation of Treg cells. The mechanism involves the stimulation of OXPHOS and the production of mROS, which leads to the increased expression of FOXP3 by increasing the levels of histone (H3K27) acetylation in the Foxp3 promoter (Hang et al., 2019). In the colon specifically, BAs act through the BA receptor Breg to regulate the function of RORg+ Treg cells, which are significant players in the maintenance of colonic homeostasis (Song et al., 2020). B Cells B cell differentiation into plasma cells and the production of antibodies require a massive amount of energy and a global change in cellular metabolism. Gut microbiota-derived SCFAs contribute to fuel the cellular energy engine at different levels for these processes and to boost antibody production. SCFAs are converted into acetyl-CoA that is integrated into the mitochondrial TCA cycle leading to the production of ATP. SCFAs also stimulate glycolysis in B cell via mTOR activation. SCFA-derived acetylCoA is also a substrate in FA (particularly palmitic acid) synthesis, which is crucial for plasma cell differentiation and stimulates antibody production (Kim et al., 2016). Using an elegant strategy based on genetically engineered Clostridium sporogenes in germ-free mice, it has recently been shown that branched SCFAs, such as isobutyrate or isovalerate, can also modulate B cell functions. The absence of branched SCFA production in manipulated mice led to an increased frequency of IgA+ plasma cells in the small intestine, and increased levels of IgA bound to the surface of innate immune cells such as neutrophils, macrophages, and dendritic cells (Guo et al., 2019). The mechanisms underlying these effects are not yet known. B cells have a critical role in tolerance toward the gut microbiota through the production of immunoglobulins and the action of IL-10-producing Bregs (regulatory B cells). In Bregs, Rosser and colleagues recently showed that butyrate could divert tryptophan metabolism toward the serotonin pathway and the production of 5-hydroxyindole-3-acetic acid (5-HIAA) (Rosser et al., 2020). Surprisingly, 5-HIAA was shown to activate AhR in these cells, mediating the suppressive effect of butyrate supplementation in a rheumatoid arthritis model in vivo. CONSEQUENCES FOR DISEASE PATHOGENESIS Immunometabolism at steady state promotes homeostasis. However, the energy requirement of immune cells during inflammatory and infectious diseases is much higher, and their whole metabolism is altered. These processes are involved in both the pathogenesis of nonseptic inflammatory disorders and in the resolution of infection (Zmora et al., 2017). As seen above, the gut microbiota modulates immunometabolism and thus can have positive or negative effects on these pathological events (Figure 3). Infections Innate immune cells are the first bulwark against bacterial infection. TCRgd (T cell receptor) IELs are key players in the initial response to intestinal pathogens. Their location within the intestinal epithelium and their motility, which are dependent on the gut microbiota, allow effective surveillance of the mucosal surface (Hoytema van Konijnenburg et al., 2017). Upon infection with Salmonella, the change in gd IEL behavior is associated with the activation of OXPHOS and anaerobic glycolysis. These metabolic changes are dependent on mTOR and microbial cues in IECs. These data highlight a complex 3-partner system in which the gut microbiota, through action on IECs, induces the metabolic reprogramming of gd IELs to boost their mucosal surveillance capacity (Hoytema van Konijnenburg et al., 2017). Metabolic changes are also observed in IECs in response to infection. In the early steps of infection with the mouse pathogen Citrobacter rodentium, downregulation of the TCA cycle and OXPHOS is observed in parallel with perturbations of cholesterol homeostasis. Cholesterol synthesis and import are activated simultaneously with cholesterol efflux, suggesting either an Figure 2. Mechanism of Activation of the mTOR Pathway by SCFAs (A) Through their action on energy production pathways, SCFAs induce the production of ATP and the depletion of AMP, which are inhibitor and activator of AMP-activated protein kinase (AMPK), respectively. Consequently, the inhibitor activity of AMPK on mTOR is repressed, thus leading to mTOR activation. (B) SCFAs, in association with P300/CBP, promote acetylation of the ribosomal protein S6 kinase beta-1 (S6K1), which is a downstream target of mTOR, leading to more robust activation of the pathway with RPS6 (ribosomal protein S6) phosphorylation and inhibition of 4EBP (translation initiation factor 4E binding protein) phosphorylation. ULK1, Unc-51 like autophagy activating kinase 1. ll 518 Cell Metabolism 32, October 6, 2020 Perspective
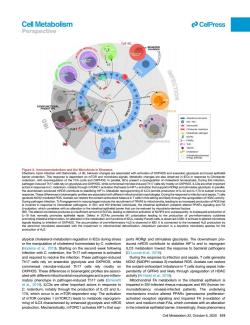
Cell Metabolism CelPress Perspective TY6 IE ● PHAGE is obse 9).Starting on econd weel to infection and sepsis,Tcellsg erate on e can restor e diffe bioen pendently of GPR43 and likely through upregulation of HDAC ated wit L,2019.c8 sm in the intest ithelium e othe important ac mpaired in SIV-infected rhesus mac es and HI human im r;I ZA which r in The 22 patients.The mplex 1 (mTORC1)leads to meta activated r tor)signaling and impa red FA Cell Metabolism 32.October 6,2020 519
atypical cholesterol metabolism regulation in IECs during stress or the manipulation of cholesterol homeostasis by C. rodentium (Hopkins et al., 2019). Starting on the second week following infection with C. rodentium, the Th17 cell response is activated and required to resolve the infection. These pathogen-induced Th17 cells rely on anaerobic glycolysis and OXPHOS, while commensal microbe-induced Th17 cells rely mostly on OXPHOS. These differences in bioenergetic profiles are associated with different mitochondrial morphologies and a pro-inflammatory phenotype in pathogen-induced Th17 cells (Omenetti et al., 2019). ILC3s are other important actors in response to C. rodentium, notably through the production of IL-22 and IL- 17A, which occur in an mTOR-dependent way. The activation of mTOR complex 1 (mTORC1) leads to metabolic reprogramming of ILC3 characterized by enhanced glycolysis and mROS production. Mechanistically, mTORC1 activates HIF1a that supports RORgt and stimulates glycolysis. The downstream produced mROS contribute to stabilize HIF1a and to reprogram ILC3 metabolism toward the response to bacterial pathogens (Di Luccia et al., 2019). During the response to infection and sepsis, T cells generate NOX2 (NADPH oxidase 2)-mediated ROS. Acetate can restore the oxidant-antioxidant imbalance in T cells during sepsis independently of GPR43 and likely through upregulation of HDAC activity (Al-Harbi et al., 2018). Mitochondrial FA metabolism in the intestinal epithelium is impaired in SIV-infected rhesus macaques and HIV (human immunodeficiency viruses)-infected patients. The underlying mechanisms involve altered PPARa (peroxisome proliferatoractivated receptor) signaling and impaired FA b-oxidation of short- and medium-chain FAs, which correlate with an alteration in the intestinal epithelial barrier. Interestingly, these phenomena Figure 3. Immunometabolism and the Microbiota in Diseases Infections. Upon infection with Salmonella, gd IEL behavior changes are associated with activation of OXPHOS and anaerobic glycolysis and boost epithelial barrier protection. This response is dependent on mTOR and microbiota signals. Metabolic changes are also observed in IECs in response to Citrobacter rodentium, with downregulation of the TCA cycle and OXPHOS. In parallel, IECs present a dysregulation of cholesterol homeostasis. During this infection, pathogen-induced Th17 cells rely on glycolysis and OXPHOS, while commensal microbe-induced Th17 cells rely mostly on OXPHOS. ILC3s are other important actors in response to C. rodentium, notably through mTORC1 activation that leads to HIF1a activation that supports RORgt and stimulates glycolysis. In parallel, the downstream produced mROS contribute to stabilizing HIF1a. Metabolic reprogramming of ILC3 permits production of IL-22 and IL-17A to sustain immune response. These differences in bioenergetic profiles are associated with different mitochondrial morphologies. During the response to infection and sepsis, T cells generate NOX2-mediated ROS. Acetate can restore the oxidant-antioxidant balance in T cells in this setting and likely through the upregulation of HDAC activity. During pathogen infection, TLR engagement in macrophages induces the recruitment of TRAF6 to mitochondria, leading to an increased production of ROS that is involved in response to intracellular pathogens. In SIV- and HIV-infected individuals, the intestinal epithelium presents altered PPARa signaling and FA b-oxidation, which correlates with an alteration in the intestinal epithelial barrier that can be restored by microbiota-derived factors. IBD. The altered microbiota produces an insufficient amount of SCFAs, leading to defective activation of NLRP3 and, subsequently, to inadequate production of IL-18 that normally promotes epithelial repair. Defect in SCFAs promotes M1 polarization leading to the production of pro-inflammatory cytokines promoting intestinal inflammation. An alteration in the metabolism and functions of IECs, notably Paneth cells, is observed in IBD. It is linked to altered microbiota signals leading to inhibition of OXPHOS. The accumulation of pro-inflammatory H2S is observed in IBD. It is connected to the increased H2S production by the abnormal microbiota associated with the impairment in mitochondrial detoxification. Atopobium parvulum is a keystone microbiota species for the production of H2S. ll Cell Metabolism 32, October 6, 2020 519 Perspective

CellPress Cell Metabolism Perspective are modulated by the gut microbiome,as mitochondrial FA severity (Mottawea et al,21).Overall,the net increase in enag0mgncepenoentotanyeiectomco4tTceiseakoe tion pathogenesis CONCLUSION ory B iota in t of the qu el dis. e (RD)has bee st-microhe intera tion al mou s in the on a hos eptor.D pite the role of the s of IEC in th mou the ules resuting in overactivation of the gut imn cts it remain scarce Yet the gut nicrobiota the gut m EC tabolis nal in Nod-like receptor (NLR)family. (nuc irge par of genome with bacteria a,so con on aor ciated N ontainingX1) effects in colitis sti et al. 2018).NLRX cell anc gut microbiota in IECs anisms are not cle nalian meta-o dulat TCA that NI RX1 may support the glutami d by or depends on metabolites fro The impaired glutami meta eads changes AA ava iity for the nmune a o 6 de metabolites are en rep montrategbgtecalmicoootararne any As induce the acti ion of NLRP3(NOD- epto rom the action of the microbiota or epithe air ah l -18ma the the cell types intr sic dive for by antibiotics,favors an M1 esp se int s and to the r inte inal in the idir nact of l,2018 that of the partner in a link he on th and dise The actionable metabolic HB1 several for modulatic n of the gu Mo one possibility.Ho ver.an even more attractive strategy is to eth c host-microbio me y simultaneously on both sides of the interking- dom cros ACKNOWLEDGMENTS t al ochondrial ment in Crohn's fH.S. es is t d in the au AUTHOR CONTRIBUTIONS The amour microbiota 5 C.M.and H.S.wrote the pap 520 Cell Metabolism 32,October6,2020
are modulated by the gut microbiome, as mitochondrial FA metabolism and intestinal barrier function can be rapidly restored by the administration of the probiotic Lactobacillus plantarum, independent of any effect on CD4+ T cells (Crakes et al., 2019). Inflammatory Bowel Disease The prominent role of the gut microbiota in the pathogenesis of inflammatory bowel disease (IBD) has been demonstrated by both human and animal studies (Britton et al., 2019; Lavelle and Sokol, 2020). The first actors in the interaction with the gut microbiota in IBD are epithelial cells. Alterations in the metabolism and functions of IECs are involved in IBD and lead to an impaired intestinal barrier and the translocation of microbial molecules, resulting in overactivation of the gut immune system. Some studies are now linking the gut microbiota to defective IEC metabolism in intestinal inflammation, notably through the Nod-like receptor (NLR) family. NLRX1 (nucleotide-binding oligomerization domain, leucine-rich repeat containing X1) is a mitochondria-associated NLR with potential anti-inflammatory effects in colitis settings (Leber et al., 2018). NLRX1 is required to maintain balanced glutamine metabolism and barrier functions in IECs. The mechanisms are not clearly demonstrated, but it is suggested that NLRX1 may support the glutamine input into the TCA cycle through its metabolism into glutamate and a-ketoglutarate. The impaired glutamine metabolism in IECs leads to changes in AA availability for the gut microbiota, inducing changes in composition. Interestingly, the altered gut microbiota exhibits a pro-inflammatory effect by itself, as demonstrated by fecal microbiota transfer experiments (Leber et al., 2018). NLR-associated inflammasomes are also involved. SCFAs induce the activation of NLRP3 (NOD-like receptor family, pyrin domain containing 3) via their receptors GPR43 and GPR109a, inducing ion (K+ and Ca2+) efflux and promoting epithelial repair in colitis setting through IL-18 maturation and release (Macia et al., 2015). The impact of SCFAs on macrophage polarization is also relevant in IBD. SCFA depletion, for example, induced by antibiotics, favors an M1 hyperresponsive phenotype leading to an overproduction of pro-inflammatory cytokines and to the promotion of intestinal inflammation (Scott et al., 2018). Previous studies have also shown a link between mitochondrial dysfunction and IBD. The expression of prohibitin 1 (PHB1), an inner mitochondrial membrane component, is decreased in colonic biopsies from IBD patients (Hsieh et al., 2006; Theiss et al., 2007). Moreover, mitochondrial dysfunction in IECs and notably in Paneth cells can induce ileal inflammation in mouse models (Jackson et al., 2020). Interestingly, Paneth cell abnormalities in patients with Crohn’s disease correlate with alterations in both microbiota composition and OXPHOS in ileal tissue (Liu et al., 2016). Mechanistically, mitochondrial respiration impairment forces IECs to acquire a dysfunctional Paneth cell phenotype, leading to metabolic imbalance and inflammation (Khaloian et al., 2020). Moreover, mitochondrial impairment in Crohn’s patients also involves a decrease in H2S detoxification, while the relative abundance of H2S-producing microbes is increased in the gut microbiota. The amount of Atopobium parvulum, a keystone microbiota species for H2S production, correlated with Crohn’s disease severity (Mottawea et al., 2016). Overall, the net increase in H2S due to increased microbiota production and decreased mitochondrial detoxification is involved in intestinal inflammation pathogenesis. CONCLUSION The effects of the gut microbiome on host immune cells are often examined with classical host-microbe interaction concepts, relying on the recognition of conserved microbial motifs by innate immunity sensors, or on the effect of microbial molecules on a host cell receptor. Despite the crucial role of the cellular metabolism in the ability to mount an appropriate immune response, the studies investigating how the gut microbiota directly affects it remain scarce. Yet the gut microbiota has a special relationship with metabolism, notably via the mitochondria due to their common origin. Mitochondria share a large part of their genome with bacteria, so communication and regulation can be evoked between these entities, which are only separated by the cell membrane (Lin and Wang, 2017). Host cell and gut microbiota are tightly connected in an inter-kingdom metabolic network that allows the proper functioning of mammalian meta-organisms. Each pathway is modulated by or depends on metabolites from others. It takes the collapse of only one path to compromise the normal operation. These processes are even more critical for immunometabolism, as immune cells need to react to stimuli rapidly and to reprogram their metabolism to exercise their functions. Gut microbiota-derived metabolites are genuinely represented in immunometabolism, with a particularly important role of SCFAs, BAs, and AA metabolites. Deciphering all the ins and outs resulting from the action of the microbiota on immunometabolism is highly challenging. Part of the complexity lies in the final effects of the microbial products, which can be different depending on the context or the cell types. The intrinsic diversity of the actors within the gut microbiota and the immune system brings an additional level of difficulty in the exploration of these interactions. The next step in the understanding of host-microbiota crosstalk is to decipher more precisely the bidirectional impact of each metabolism on that of the partner in health and disease. This effort is crucial to identify therapeutic targets that will be actionable through metabolic modulation. These innovative treatments may take several forms. The modulation of the gut microbiota to favor beneficial metabolite-producing bacteria is one possibility. However, an even more attractive strategy is to precisely impact host-microbiota metabolism by accurately supplementing a missing metabolite and/or inhibiting an overactivated pathway simultaneously on both sides of the interkingdom crosstalk. ACKNOWLEDGMENTS H.S. received funding from the European Research Council (ERC) under the European Union’s Horizon 2020 Research and Innovation Programme (ERC- 2016-StG-71577). We thank Hugo Michaudel for the design of the figures. AUTHOR CONTRIBUTIONS C.M. and H.S. wrote the paper. ll 520 Cell Metabolism 32, October 6, 2020 Perspective
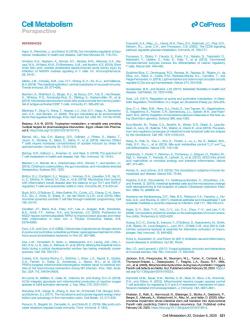
Cell Metabolism CelPress Perspective REFERENCES M(2017)Metabolic eyn health and SoCsane智aonovo8edPdaeP9eo2sg optimal function.Immunity131-132 474778 eeataggmcrobotanaapiemmuneho io B20990Bn,8。co o.Cell 166376 hoo y.A.H(20) caroeoramhesnirognemd uences Cel158705-721. tmaaaeao17fa2t6gmunyaaneactone 2 Cell Metabolism 32.October 6.2020 521
REFERENCES Agus, A., Planchais, J., and Sokol, H. (2018). Gut microbiota regulation of tryptophan metabolism in health and disease. Cell Host Microbe 23, 716–724. Al-Harbi, N.O., Nadeem, A., Ahmad, S.F., Alotaibi, M.R., AlAsmari, A.F., Alanazi, W.A., Al-Harbi, M.M., El-Sherbeeny, A.M., and Ibrahim, K.E. (2018). Short chain fatty acid, acetate ameliorates sepsis-induced acute kidney injury by inhibition of NADPH oxidase signaling in T cells. Int. Immunopharmacol. 58, 24–31. Allaire, J.M., Crowley, S.M., Law, H.T., Chang, S.-Y., Ko, H.-J., and Vallance, B.A. (2018). The intestinal epithelium: central coordinator of mucosal immunity. Trends Immunol. 39, 677–696. Bachem, A., Makhlouf, C., Binger, K.J., de Souza, D.P., Tull, D., Hochheiser, K., Whitney, P.G., Fernandez-Ruiz, D., Dahling, S., Kastenm € uller, W., et al. € (2019). Microbiota-derived short-chain fatty acids promote the memory potential of antigen-activated CD8+ T cells. Immunity 51, 285–297.e5. Backhed, F., Ding, H., Wang, T., Hooper, L.V., Koh, G.Y., Nagy, A., Semenko- € vich, C.F., and Gordon, J.I. (2004). The gut microbiota as an environmental factor that regulates fat storage. Proc. Natl. Acad. Sci. USA 101, 15718–15723. Badawy, A.A.-B. (2019). Tryptophan metabolism: a versatile area providing multiple targets for pharmacological intervention. Egypt J Basic Clin Pharmacol 9, https://doi.org/10.32527/2019/101415. Balmer, M.L., Ma, E.H., Bantug, G.R., Grahlert, J., Pfister, S., Glatter, T., € Jauch, A., Dimeloe, S., Slack, E., Dehio, P., et al. (2016). Memory CD8(+) T cells require increased concentrations of acetate induced by stress for optimal function. Immunity 44, 1312–1324. Bantug, G.R., Galluzzi, L., Kroemer, G., and Hess, C. (2018). The spectrum of T cell metabolism in health and disease. Nat. Rev. Immunol. 18, 19–34. Blanton, L.V., Barratt, M.J., Charbonneau, M.R., Ahmed, T., and Gordon, J.I. (2016). Childhood undernutrition, the gut microbiota, and microbiota-directed therapeutics. Science 352, 1533. Britton, G.J., Contijoch, E.J., Mogno, I., Vennaro, O.H., Llewellyn, S.R., Ng, R., Li, Z., Mortha, A., Merad, M., Das, A., et al. (2019). Microbiotas from humans with inflammatory bowel disease alter the balance of gut Th17 and RORgt + regulatory T cells and exacerbate colitis in mice. Immunity 50, 212–224.e4. Buck, M.D., O’Sullivan, D., Klein Geltink, R.I., Curtis, J.D., Chang, C.-H., Sanin, D.E., Qiu, J., Kretz, O., Braas, D., van der Windt, G.J.W., et al. (2016). Mitochondrial dynamics controls T cell fate through metabolic programming. Cell 166, 63–76. Cavallari, J.F., Barra, N.G., Foley, K.P., Lee, A., Duggan, B.M., Henriksbo, B.D., Anheˆ , F.F., Ashkar, A.A., and Schertzer, J.D. (2020). Postbiotics for NOD2 require nonhematopoietic RIPK2 to improve blood glucose and metabolic inflammation in mice. Am. J. Physiol. Endocrinol. Metab. 318, E579–E585. Cory, J.G., and Cory, A.H. (2006). Critical roles of glutamine as nitrogen donors in purine and pyrimidine nucleotide synthesis: asparaginase treatment in childhood acute lymphoblastic leukemia. In Vivo 20, 587–589. Cox, L.M., Yamanishi, S., Sohn, J., Alekseyenko, A.V., Leung, J.M., Cho, I., Kim, S.G., Li, H., Gao, Z., Mahana, D., et al. (2014). Altering the intestinal microbiota during a critical developmental window has lasting metabolic consequences. Cell 158, 705–721. Crakes, K.R., Santos Rocha, C., Grishina, I., Hirao, L.A., Napoli, E., Gaulke, C.A., Fenton, A., Datta, S., Arredondo, J., Marco, M.L., et al. (2019). PPARa-targeted mitochondrial bioenergetics mediate repair of intestinal barriers at the host-microbe intersection during SIV infection. Proc. Natl. Acad. Sci. USA 116, 24819–24829. Di Luccia, B., Gilfillan, S., Cella, M., Colonna, M., and Huang, S.C.-C. (2019). ILC3s integrate glycolysis and mitochondrial production of reactive oxygen species to fulfill activation demands. J. Exp. Med. 216, 2231–2241. Donohoe, D.R., Garge, N., Zhang, X., Sun, W., O’Connell, T.M., Bunger, M.K., and Bultman, S.J. (2011). The microbiome and butyrate regulate energy metabolism and autophagy in the mammalian colon. Cell Metab. 13, 517–526. Fiorucci, S., Biagioli, M., Zampella, A., and Distrutti, E. (2018). Bile acids activated receptors regulate innate immunity. Front. Immunol. 9, 1853. Frauwirth, K.A., Riley, J.L., Harris, M.H., Parry, R.V., Rathmell, J.C., Plas, D.R., Elstrom, R.L., June, C.H., and Thompson, C.B. (2002). The CD28 signaling pathway regulates glucose metabolism. Immunity 16, 769–777. Furusawa, Y., Obata, Y., Fukuda, S., Endo, T.A., Nakato, G., Takahashi, D., Nakanishi, Y., Uetake, C., Kato, K., Kato, T., et al. (2013). Commensal microbe-derived butyrate induces the differentiation of colonic regulatory T cells. Nature 504, 446–450. Godinho-Silva, C., Domingues, R.G., Rendas, M., Raposo, B., Ribeiro, H., da Silva, J.A., Vieira, A., Costa, R.M., Barbosa-Morais, N.L., Carvalho, T., and Veiga-Fernandes, H. (2019). Light-entrained and brain-tuned circadian circuits regulate ILC3s and gut homeostasis. Nature 574, 254–258. Goodpaster, B.H., and Sparks, L.M. (2017). Metabolic flexibility in health and disease. Cell Metab. 25, 1027–1036. Gots, J.S. (1971). Regulation of purine and pyrimidine metabolism. In Metabolic Regulation, Third Edition, H.J. Vogel, ed. (Academic Press), pp. 225–255. Guo, C.-J., Allen, B.M., Hiam, K.J., Dodd, D., Van Treuren, W., Higginbottom, S., Nagashima, K., Fischer, C.R., Sonnenburg, J.L., Spitzer, M.H., and Fischbach, M.A. (2019). Depletion of microbiome-derived molecules in the host using Clostridium genetics. Science 366, eaav1282. Gury-BenAri, M., Thaiss, C.A., Serafini, N., Winter, D.R., Giladi, A., Lara-Astiaso, D., Levy, M., Salame, T.M., Weiner, A., David, E., et al. (2016). The spectrum and regulatory landscape of intestinal innate lymphoid cells are shaped by the microbiome. Cell 166, 1231–1246.e13. Hang, S., Paik, D., Yao, L., Kim, E., Trinath, J., Lu, J., Ha, S., Nelson, B.N., Kelly, S.P., Wu, L., et al. (2019). Bile acid metabolites control TH17 and Treg cell differentiation. Nature 576, 143–148. Hashimoto, T., Perlot, T., Rehman, A., Trichereau, J., Ishiguro, H., Paolino, M., Sigl, V., Hanada, T., Hanada, R., Lipinski, S., et al. (2012). ACE2 links amino acid malnutrition to microbial ecology and intestinal inflammation. Nature 487, 477–481. Honda, K., and Littman, D.R. (2016). The microbiota in adaptive immune homeostasis and disease. Nature 535, 75–84. Hopkins, E.G.D., Roumeliotis, T.I., Mullineaux-Sanders, C., Choudhary, J.S., and Frankel, G. (2019). Intestinal epithelial cells and the microbiome undergo swift reprogramming at the inception of colonic Citrobacter rodentium infection. MBio 10, e00062-19. Hoytema van Konijnenburg, D.P., Reis, B.S., Pedicord, V.A., Farache, J., Victora, G.D., and Mucida, D. (2017). Intestinal epithelial and intraepithelial T cell crosstalk mediates a dynamic response to infection. Cell 171, 783–794.e13. Hsieh, S.-Y., Shih, T.-C., Yeh, C.-Y., Lin, C.-J., Chou, Y.-Y., and Lee, Y.-S. (2006). Comparative proteomic studies on the pathogenesis of human ulcerative colitis. Proteomics 6, 5322–5331. Huang, S.C.-C., Everts, B., Ivanova, Y., O’Sullivan, D., Nascimento, M., Smith, A.M., Beatty, W., Love-Gregory, L., Lam, W.Y., O’Neill, C.M., et al. (2014). Cellintrinsic lysosomal lipolysis is essential for alternative activation of macrophages. Nat. Immunol. 15, 846–855. Hviid, A., Svanstro¨ m, H., and Frisch, M. (2011). Antibiotic use and inflammatory bowel diseases in childhood. Gut 60, 49–54. Iliev, I.D., and Leonardi, I. (2017). Fungal dysbiosis: immunity and interactions at mucosal barriers. Nat. Rev. Immunol. 17, 635–646. Jackson, D.N., Panopoulos, M., Neumann, W.L., Turner, K., Cantarel, B.L., Thompson-Snipes, L., Dassopoulos, T., Feagins, L.A., Souza, R.F., Mills, J.C., et al. (2020). Mitochondrial dysfunction during loss of prohibitin 1 triggers Paneth cell defects and ileitis. Gut. Published online February 28, 2020. https:// doi.org/10.1136/gutjnl-2019-319523. Kaminski, M.M., Sauer, S.W., Klemke, C.-D., Suss, D., Okun, J.G., Krammer, € P.H., and Gulow, K. (2010). Mitochondrial reactive oxygen species control € T cell activation by regulating IL-2 and IL-4 expression: mechanism of cipro- floxacin-mediated immunosuppression. J. Immunol. 184, 4827–4841. Khaloian, S., Rath, E., Hammoudi, N., Gleisinger, E., Blutke, A., Giesbertz, P., Berger, E., Metwaly, A., Waldschmitt, N., Allez, M., and Haller, D. (2020). Mitochondrial impairment drives intestinal stem cell transition into dysfunctional Paneth cells predicting Crohn’s disease recurrence. Gut. Published online February 28, 2020. https://doi.org/10.1136/gutjnl-2019-319514. ll Cell Metabolism 32, October 6, 2020 521 Perspective

CelPress Cell Metabolism Perspective ho metaboes ,ta201.a oetMsn8SmCf82.uhgwhyoursetmnateymphodce microblota.metabolites and hos cvtonCel Metap1. Hg89oo0tm呼 10. ShikoporovAand Hll CBactenophge of me t,M.R..Panikov.N..Michaud.M..Gatni.CA Bohlooly-' e'h0eetaboieaheratonsasociaedwhagninedeficien osstalk in n's dis te out ROR·requlatory T ce 2oe,296e紫ommrone tA66a登eoRh
Kim, M., Qie, Y., Park, J., and Kim, C.H. (2016). Gut microbial metabolites fuel host antibody responses. Cell Host Microbe 20, 202–214. Klose, C.S.N., and Artis, D. (2016). Innate lymphoid cells as regulators of immunity, inflammation and tissue homeostasis. Nat. Immunol. 17, 765–774. Lampropoulou, V., Sergushichev, A., Bambouskova, M., Nair, S., Vincent, E.E., Loginicheva, E., Cervantes-Barragan, L., Ma, X., Huang, S.C.-C., Griss, T., et al. (2016). Itaconate links inhibition of succinate dehydrogenase with macrophage metabolic remodeling and regulation of inflammation. Cell Metab. 24, 158–166. Lavelle, A., and Sokol, H. (2020). Gut microbiota-derived metabolites as key actors in inflammatory bowel disease. Nat. Rev. Gastroenterol. Hepatol. 17, 223–237. Leber, A., Hontecillas, R., Tubau-Juni, N., Zoccoli-Rodriguez, V., Abedi, V., and Bassaganya-Riera, J. (2018). NLRX1 modulates immunometabolic mechanisms controlling the host-gut microbiota interactions during inflammatory bowel disease. Front. Immunol. 9, 363. Lewis, G., Wang, B., Shafiei Jahani, P., Hurrell, B.P., Banie, H., Aleman Muench, G.R., Maazi, H., Helou, D.G., Howard, E., Galle-Treger, L., et al. (2019). Dietary fiber-induced microbial short chain fatty acids suppress ILC2-dependent airway inflammation. Front. Immunol. 10, 2051. Lin, C.J., and Wang, M.C. (2017). Microbial metabolites regulate host lipid metabolism through NR5A-Hedgehog signalling. Nat. Cell Biol. 19, 550–557. Liu, T.-C., Gurram, B., Baldridge, M.T., Head, R., Lam, V., Luo, C., Cao, Y., Simpson, P., Hayward, M., Holtz, M.L., et al. (2016). Paneth cell defects in Crohn’s disease patients promote dysbiosis. JCI Insight 1, e86907. Liu, Y., Hou, Y., Wang, G., Zheng, X., and Hao, H. (2020). Gut microbial metabolites of aromatic amino acids as signals in host-microbe interplay. Trends Endocrinol. Metab. Published online April 10, 2020. https://doi.org/10.1016/ j.tem.2020.02.012. Lunt, S.Y., and Vander Heiden, M.G. (2011). Aerobic glycolysis: meeting the metabolic requirements of cell proliferation. Annu. Rev. Cell Dev. Biol. 27, 441–464. Luu, M., Pautz, S., Kohl, V., Singh, R., Romero, R., Lucas, S., Hofmann, J., Raifer, H., Vachharajani, N., Carrascosa, L.C., et al. (2019). The short-chain fatty acid pentanoate suppresses autoimmunity by modulating the metabolicepigenetic crosstalk in lymphocytes. Nat. Commun. 10, 760. Macia, L., Tan, J., Vieira, A.T., Leach, K., Stanley, D., Luong, S., Maruya, M., Ian McKenzie, C., Hijikata, A., Wong, C., et al. (2015). Metabolite-sensing receptors GPR43 and GPR109A facilitate dietary fibre-induced gut homeostasis through regulation of the inflammasome. Nat. Commun. 6, 6734. Mao, K., Baptista, A.P., Tamoutounour, S., Zhuang, L., Bouladoux, N., Martins, A.J., Huang, Y., Gerner, M.Y., Belkaid, Y., and Germain, R.N. (2018). Innate and adaptive lymphocytes sequentially shape the gut microbiota and lipid metabolism. Nature 554, 255–259. Martin-Gallausiaux, C., Larraufie, P., Jarry, A., Be´ guet-Crespel, F., Marinelli, L., Ledue, F., Reimann, F., Blottie`re, H.M., and Lapaque, N. (2018). Butyrate produced by commensal bacteria down-regulates Indolamine 2,3-Dioxygenase 1 (IDO-1) expression via a dual mechanism in human intestinal epithelial cells. Front. Immunol. 9, 2838. Mills, E.L., Kelly, B., Logan, A., Costa, A.S.H., Varma, M., Bryant, C.E., Tourlomousis, P., Dabritz, J.H.M., Gottlieb, E., Latorre, I., et al. (2016). Succinate € dehydrogenase supports metabolic repurposing of mitochondria to drive in- flammatory macrophages. Cell 167, 457–470.e13. Milner, J.A. (1985). Metabolic aberrations associated with arginine deficiency. J. Nutr. 115, 516–523. Mottawea, W., Chiang, C.-K., Muhlbauer, M., Starr, A.E., Butcher, J., Abuja- € mel, T., Deeke, S.A., Brandel, A., Zhou, H., Shokralla, S., et al. (2016). Altered intestinal microbiota-host mitochondria crosstalk in new onset Crohn’s disease. Nat. Commun. 7, 13419. O’Neill, L.A.J., Kishton, R.J., and Rathmell, J. (2016). A guide to immunometabolism for immunologists. Nat. Rev. Immunol. 16, 553–565. Omenetti, S., Bussi, C., Metidji, A., Iseppon, A., Lee, S., Tolaini, M., Li, Y., Kelly, G., Chakravarty, P., Shoaie, S., et al. (2019). The intestine harbors functionally distinct homeostatic tissue-resident and inflammatory Th17 cells. Immunity 51, 77–89.e6. Park, J., Kim, M., Kang, S.G., Jannasch, A.H., Cooper, B., Patterson, J., and Kim, C.H. (2015). Short-chain fatty acids induce both effector and regulatory T cells by suppression of histone deacetylases and regulation of the mTORS6K pathway. Mucosal Immunol. 8, 80–93. Pasolli, E., Asnicar, F., Manara, S., Zolfo, M., Karcher, N., Armanini, F., Beghini, F., Manghi, P., Tett, A., Ghensi, P., et al. (2019). Extensive unexplored human microbiome diversity revealed by over 150,000 genomes from metagenomes spanning age, geography, and lifestyle. Cell 176, 649–662.e20. Pigneur, B., and Sokol, H. (2016). Fecal microbiota transplantation in inflammatory bowel disease: the quest for the holy grail. Mucosal Immunol. 9, 1360–1365. Richard, M.L., and Sokol, H. (2019). The gut mycobiota: insights into analysis, environmental interactions and role in gastrointestinal diseases. Nat. Rev. Gastroenterol. Hepatol. 16, 331–345. Rodrı´guez-Prados, J.-C., Trave´ s, P.G., Cuenca, J., Rico, D., Aragone´ s, J., Martı´n-Sanz, P., Cascante, M., and Bosca´ , L. (2010). Substrate fate in activated macrophages: a comparison between innate, classic, and alternative activation. J. Immunol. 185, 605–614. Rolot, M., and O’Sullivan, T.E. (2020). Living with yourself: innate lymphoid cell immunometabolism. Cells 9, E334. Rooks, M.G., and Garrett, W.S. (2016). Gut microbiota, metabolites and host immunity. Nat. Rev. Immunol. 16, 341–352. Rosser, E.C., Piper, C.J.M., Matei, D.E., Blair, P.A., Rendeiro, A.F., Orford, M., Alber, D.G., Krausgruber, T., Catalan, D., Klein, N., et al. (2020). Microbiotaderived metabolites suppress arthritis by amplifying aryl-hydrocarbon receptor activation in regulatory B cells. Cell Metab. 31, 837–851.e10. Sarin, S.K., Pande, A., and Schnabl, B. (2019). Microbiome as a therapeutic target in alcohol-related liver disease. J. Hepatol. 70, 260–272. Schneider, C., O’Leary, C.E., von Moltke, J., Liang, H.-E., Ang, Q.Y., Turnbaugh, P.J., Radhakrishnan, S., Pellizzon, M., Ma, A., and Locksley, R.M. (2018). A metabolite-triggered tuft cell-ILC2 circuit drives small intestinal remodeling. Cell 174, 271–284.e14. Scott, N.A., Andrusaite, A., Andersen, P., Lawson, M., Alcon-Giner, C., Leclaire, C., Caim, S., Le Gall, G., Shaw, T., Connolly, J.P.R., et al. (2018). Antibiotics induce sustained dysregulation of intestinal T cell immunity by perturbing macrophage homeostasis. Sci. Transl. Med. 10, eaao4755. Sena, L.A., Li, S., Jairaman, A., Prakriya, M., Ezponda, T., Hildeman, D.A., Wang, C.-R., Schumacker, P.T., Licht, J.D., Perlman, H., et al. (2013). Mitochondria are required for antigen-specific T cell activation through reactive oxygen species signaling. Immunity 38, 225–236. Sender, R., Fuchs, S., and Milo, R. (2016). Are we really vastly outnumbered? Revisiting the ratio of bacterial to host cells in humans. Cell 164, 337–340. Shajib, M.S., and Khan, W.I. (2015). The role of serotonin and its receptors in activation of immune responses and inflammation. Acta Physiol. (Oxf.) 213, 561–574. Shkoporov, A.N., and Hill, C. (2019). Bacteriophages of the human gut: the ‘‘known unknown’’ of the microbiome. Cell Host Microbe 25, 195–209. Skelly, A.N., Sato, Y., Kearney, S., and Honda, K. (2019). Mining the microbiota for microbial and metabolite-based immunotherapies. Nat. Rev. Immunol. 19, 305–323. Smith, P.M., Howitt, M.R., Panikov, N., Michaud, M., Gallini, C.A., Bohlooly-Y, M., Glickman, J.N., and Garrett, W.S. (2013). The microbial metabolites, shortchain fatty acids, regulate colonic Treg cell homeostasis. Science 341, 569–573. Song, X., Sun, X., Oh, S.F., Wu, M., Zhang, Y., Zheng, W., Geva-Zatorsky, N., Jupp, R., Mathis, D., Benoist, C., and Kasper, D.L. (2020). Microbial bile acid metabolites modulate gut RORg+ regulatory T cell homeostasis. Nature 577, 410–415. Tannahill, G.M., Curtis, A.M., Adamik, J., Palsson-McDermott, E.M., McGettrick, A.F., Goel, G., Frezza, C., Bernard, N.J., Kelly, B., Foley, N.H., et al. (2013). Succinate is an inflammatory signal that induces IL-1b through HIF-1a. Nature 496, 238–242. Thaiss, C.A., Levy, M., Korem, T., Dohnalova´ , L., Shapiro, H., Jaitin, D.A., David, E., Winter, D.R., Gury-BenAri, M., Tatirovsky, E., et al. (2016). Microbiota ll 522 Cell Metabolism 32, October 6, 2020 Perspective
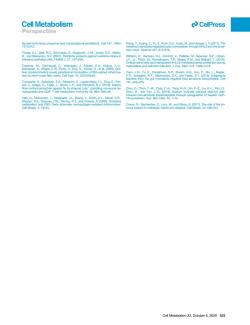
Cell Metabolism CelPress Perspective i02al2hamicyprogramshostansciptomeoscaitons.Cal767,149s- ILC2- ob8neamve8配0aeease70282a8i880-eaioaniama maNicod nd Msnd Dtary Zhou,D.Chen,Y.-W..Zhao H.Yang.R.X.Xin.F..Liu.XPan.O. 折eioes6a0kwae2oughpegu6ionoiepa Cell Metab.413-24. Cell Metabolism 32,October 6,2020 523
diurnal rhythmicity programs host transcriptome oscillations. Cell 167, 1495– 1510.e12. Theiss, A.L., Idell, R.D., Srinivasan, S., Klapproth, J.-M., Jones, D.P., Merlin, D., and Sitaraman, S.V. (2007). Prohibitin protects against oxidative stress in intestinal epithelial cells. FASEB J. 21, 197–206. Trapecar, M., Communal, C., Velazquez, J., Maass, C.A., Huang, Y.-J., Schneider, K., Wright, C.W., Butty, V., Eng, G., Yilmaz, O., et al. (2020). Gutliver physiomimetics reveal paradoxical modulation of IBD-related inflammation by short-chain fatty acids. Cell Syst. 10, 223–239.e9. Trompette, A., Gollwitzer, E.S., Pattaroni, C., Lopez-Mejia, I.C., Riva, E., Pernot, J., Ubags, N., Fajas, L., Nicod, L.P., and Marsland, B.J. (2018). Dietary fiber confers protection against flu by shaping Ly6c patrolling monocyte hematopoiesis and CD8+ T cell metabolism. Immunity 48, 992–1005.e8. Vats, D., Mukundan, L., Odegaard, J.I., Zhang, L., Smith, K.L., Morel, C.R., Wagner, R.A., Greaves, D.R., Murray, P.J., and Chawla, A. (2006). Oxidative metabolism and PGC-1beta attenuate macrophage-mediated inflammation. Cell Metab. 4, 13–24. Wang, Y., Kuang, Z., Yu, X., Ruhn, K.A., Kubo, M., and Hooper, L.V. (2017). The intestinal microbiota regulates body composition through NFIL3 and the circadian clock. Science 357, 912–916. Wilhelm, C., Harrison, O.J., Schmitt, V., Pelletier, M., Spencer, S.P., Urban, J.F., Jr., Ploch, M., Ramalingam, T.R., Siegel, R.M., and Belkaid, Y. (2016). Critical role of fatty acid metabolism in ILC2-mediated barrier protection during malnutrition and helminth infection. J. Exp. Med. 213, 1409–1418. Yano, J.M., Yu, K., Donaldson, G.P., Shastri, G.G., Ann, P., Ma, L., Nagler, C.R., Ismagilov, R.F., Mazmanian, S.K., and Hsiao, E.Y. (2015). Indigenous bacteria from the gut microbiota regulate host serotonin biosynthesis. Cell 161, 264–276. Zhou, D., Chen, Y.-W., Zhao, Z.-H., Yang, R.-X., Xin, F.-Z., Liu, X.-L., Pan, Q., Zhou, H., and Fan, J.-G. (2018). Sodium butyrate reduces high-fat dietinduced non-alcoholic steatohepatitis through upregulation of hepatic GLP- 1R expression. Exp. Mol. Med. 50, 1–12. Zmora, N., Bashiardes, S., Levy, M., and Elinav, E. (2017). The role of the immune system in metabolic health and disease. Cell Metab. 25, 506–521. ll Cell Metabolism 32, October 6, 2020 523 Perspective
按次数下载不扣除下载券;
注册用户24小时内重复下载只扣除一次;
顺序:VIP每日次数-->可用次数-->下载券;
- 南方医科大学:《生物化学》课程教学资源(PPT课件讲稿)读内经、学养生.pptx
- 南方医科大学:《生物化学》课程教学资源(PPT课件讲稿)分子生物学——肠道健康管理.pptx
- 南方医科大学:《生物化学》课程教学资源(PPT课件讲稿)分子生物学——解码基因、健康生活.ppt
- 南方医科大学:《生物化学》课程教学资源(PPT课件讲稿)分子生物学应用概述.pptx
- 南方医科大学:《生物化学》课程教学资源(PPT课件讲稿)血液生物化学 Hemal Biochemistry.pptx
- 南方医科大学:《生物化学》课程教学资源(PPT课件讲稿)肝脏生化(肝的生物化学 Biochemistry in Liver).pptx
- 南方医科大学:《生物化学》课程教学资源(PPT课件讲稿)代谢整合与调节 Integration and Regulation of Metabolism.pptx
- 南方医科大学:《生物化学》课程教学资源(PPT课件讲稿)脂质代谢 Metabolism of Lipids.pptx
- 南方医科大学:《生物化学》课程教学资源(PPT课件讲稿)生物氧化 Biological oxidation.pptx
- 南方医科大学:《生物化学》课程教学资源(PPT课件讲稿)氨基酸代谢 Metabolism of Amino Acids.pptx
- 南方医科大学:《生物化学》课程教学资源(PPT课件讲稿)核酸的结构与功能 Structure and Function of Nucleic Acid(主讲:彭翼飞).ppt
- 南方医科大学:《生物化学》课程教学资源(PPT课件讲稿)核苷酸代谢 Metabolism of Nucleotides(主讲:朱利娜).pptx
- 《生物化学》课程教学资源(书籍文献)经典教材 Lehninger Principles of Biochemistry, SIXTH EDITION, David L. Nelson, Michael M. Cox.pdf
- 《生物化学》课程教学资源(书籍文献)英文生化教材 Lippincott’s Illustrated Reviews Biochemistry, 5th 2010, Richard A. Harvey, PhD, Denise R. Ferrier, PhD.pdf
- 《生物化学》课程教学资源(书籍文献)Biochemistry, EIGHTH EDITION 2015, Jeremy M. Berg, John L. Tymoczko, Gregory J. Gatto, Jr. Lubert Stryer.pdf
- 《生物化学》课程教学资源(书籍文献)Biochemistry, sixth edition 2017, Reginald H. Garrett, Charles M. Grisham, University of Virginia.pdf
- 山东第一医科大学(泰山医学院):《生理学》课程教学资源(PPT课件)第十二章 生殖(2/2)第二节 女性生殖.ppt
- 山东第一医科大学(泰山医学院):《生理学》课程教学资源(PPT课件)第十二章 生殖(1/2)第一节 男性生殖.ppt
- 山东第一医科大学(泰山医学院):《生理学》课程教学资源(PPT课件)第十一章 内分泌(3/3)第六节 肾上腺的内分泌.pptx
- 山东第一医科大学(泰山医学院):《生理学》课程教学资源(PPT课件)第十章 神经系统(3/3).ppt
- 《生物化学》课程教学资源(文献资料)常用分子生物学技术——主要基因检测方法原理及优缺点.docx
- 南方医科大学:《生物化学》课程教学资源(PPT课件讲稿)DNA重组与重组DNA技术.pptx
- 南方医科大学:《生物化学》课程教学资源(PPT课件讲稿)癌基因与抑癌基因 Oncogenes, Tumor suppressor gene.ppt
- 南方医科大学:《生物化学》课程教学资源(PPT课件讲稿)基因与疾病的关系 GENE AND DISEASE.ppt
- 南方医科大学:《生物化学》课程教学资源(PPT课件讲稿)基因诊断 Gene Diagnosis.ppt
- 南方医科大学:《生物化学》课程教学资源(讲义)液态活检.docx
- 南方医科大学:《生物化学》课程教学资源(讲义)非小细胞肺癌的靶向药物治疗.docx
- 《生物化学》课程教学资源(讲义)三种基因编辑工具的比较.docx
- 南方医科大学:《生物化学》课程教学资源(PPT课件讲稿)基因治疗(主讲:宋艳斌).ppt
- 南方医科大学:《生物化学》课程教学资源(PPT课件讲稿)基因编辑技术.pptx
- 《生物化学》课程教学资源(文献资料)非小细胞肺癌和EGFR.pdf
- 《生物化学》课程教学资源(文献资料)新冠病毒——病例分析.pdf
- 《生物化学》课程教学资源(教学案例)新冠肺炎.docx
- 南方医科大学:《生物化学》课程教学资源(教学案例)地中海贫血.docx
- 《生物化学》课程教学资源(文献资料)新冠病毒奥密克戎(Omicron, B.1.1.529)变异株对我市影响的风险评估及防控建议.pdf
- 牡丹江医学院:《病原生物学》课程PPT教学课件(讲稿)医学免疫学实验.ppt
- 《医学免疫学》课程PPT教学课件(讲稿)形态学研究及其方法进展.ppt
- 南京医科大学:生物芯片技术及其应用(PPT课件讲稿,主讲:童明庆).ppt
- 上海市肿瘤研究所:肿瘤问题(PPT讲稿)肿瘤分子生物学前沿的若干问题.ppt
- 吉林大学:《病理生理学》课程教学大纲(研究生)病理生理学 Syllabus of Pathophysiology.pdf