《环境微生物学与实验》课程教学资源(文献资料)Microbial Nutrition

thoc PART I CHAPTER 5 Microbial Microbial Nutrition Nutrition Growth,and Control Chapter 5 Microbial Nutrition Chapter 6 Microbial Growth Chapter 7 Control of Microorganisms by Physical and Chemical Agents Outline Concepts 5.1 The Comm 1.Mic p 53 54 ts by the 103 104 7 on,waler an .8 108
Prescott−Harley−Klein: Microbiology, Fifth Edition II. Microbial Nutrition, Growth, and Control 5. Microbial Nutrition © The McGraw−Hill Companies, 2002 PART II Microbial Nutrition, Growth, and Control Chapter 5 Microbial Nutrition Chapter 6 Microbial Growth Chapter 7 Control of Microorganisms by Physical and Chemical Agents CHAPTER 5 Microbial Nutrition Staphylococcus aureus forms large, golden colonies when growing on blood agar. This human pathogen causes diseases such as boils, abscesses, bacteremia, endocarditis, food poisoning, pharyngitis, and pneumonia. Outline 5.1 The Common Nutrient Requirements 96 5.2 Requirements for Carbon, Hydrogen, and Oxygen 96 5.3 Nutritional Types of Microorganisms 97 5.4 Requirements for Nitrogen, Phosphorus, and Sulfur 98 5.5 Growth Factors 98 5.6Uptake of Nutrients by the Cell 100 Facilitated Diffusion 100 Active Transport 101 Group Translocation 103 Iron Uptake 104 5.7 Culture Media 104 Synthetic or Defined Media 104 Complex Media 105 Types of Media 105 5.8 Isolation of Pure Cultures 106 The Spread Plate and Streak Plate 106 The Pour Plate 107 Colony Morphology and Growth 108 Concepts 1. Microorganisms require about 10 elements in large quantities, in part because they are used to construct carbohydrates, lipids, proteins, and nucleic acids. Several other elements are needed in very small amounts and are parts of enzymes and cofactors. 2. All microorganisms can be placed in one of a few nutritional categories on the basis of their requirements for carbon, energy, and hydrogen atoms or electrons. 3. Nutrient molecules frequently cannot cross selectively permeable plasma membranes through passive diffusion. They must be transported by one of three major mechanisms involving the use of membrane carrier proteins. Eucaryotic microorganisms also employ endocytosis for nutrient uptake. 4. Culture media are needed to grow microorganisms in the laboratory and to carry out specialized procedures like microbial identification, water and food analysis, and the isolation of particular microorganisms. Many different media are available for these and other purposes. 5. Pure cultures can be obtained through the use of spread plates, streak plates, or pour plates and are required for the careful study of an individual microbial species

5.Microbial Nutrition 6 Chapter 5 Microbial Nutrition Besides the common macroelements and trace elements,n nvironment.Diatoms (see and oceans (ee pp.2. pendon the presenceo Finally.it must be emphasized that micr rganisms requir wthwill be limited rega rdless of the con on and therefo c a lor micr centrations of other nutrients. ental facte 52 of microorganisms.These cussed in chapter 6 after an introduction to micre ng as carbon sour 5.1 The Common Nutrient Requirements thes nts ced and that they ca Indeed.the more reduced .the bigher their because they nisms in relatively larg yhenthceclecions move fro reduced donor ed electron don't have to. cium (Ca)among other functions.con s to the heat resist CO,is c m送m and F)isa part of cytochromes can use CO,as thei eral otr most cells.Howe ver.cells requir aminants in water.glass reduced.comp nts are tous and prot heterotrophs (these prefor rmed molecules normally come from ociation of regulatory and catalytic subun in E coli as- ergy.For exam ple.the glycolytic pathway produces carbon skel ses energy as ATP and snhat NADH A most remarkable nutrit ristic of microo s and enz
Prescott−Harley−Klein: Microbiology, Fifth Edition II. Microbial Nutrition, Growth, and Control 5. Microbial Nutrition © The McGraw−Hill Companies, 2002 The whole of nature, as has been said, is a conjugation of the verb to eat, in the active and passive. —William Ralph Inge To obtain energy and construct new cellular components, organisms must have a supply of raw materials or nutrients. Nutrients are substances used in biosynthesis and energy production and therefore are required for microbial growth. This chapter describes the nutritional requirements of microorganisms, how nutrients are acquired, and the cultivation of microorganisms. Environmental factors such as temperature, oxygen levels, and the osmotic concentration of the medium are critical in the successful cultivation of microorganisms. These topics are discussed in chapter 6 after an introduction to microbial growth. 5.1 The Common Nutrient Requirements Analysis of microbial cell composition shows that over 95% of cell dry weight is made up of a few major elements: carbon, oxygen, hydrogen, nitrogen, sulfur, phosphorus, potassium, calcium, magnesium, and iron. These are called macroelements or macronutrients because they are required by microorganisms in relatively large amounts. The first six (C, O, H, N, S, and P) are components of carbohydrates, lipids, proteins, and nucleic acids. The remaining four macroelements exist in the cell as cations and play a variety of roles. For example, potassium (K) is required for activity by a number of enzymes, including some of those involved in protein synthesis. Calcium (Ca2), among other functions, contributes to the heat resistance of bacterial endospores. Magnesium (Mg2) serves as a cofactor for many enzymes, complexes with ATP, and stabilizes ribosomes and cell membranes. Iron (Fe2 and Fe3) is a part of cytochromes and a cofactor for enzymes and electron-carrying proteins. All organisms, including microorganisms, require several micronutrients or trace elements besides macroelements. The micronutrients—manganese, zinc, cobalt, molybdenum, nickel, and copper—are needed by most cells. However, cells require such small amounts that contaminants in water, glassware, and regular media components often are adequate for growth. Therefore it is very difficult to demonstrate a micronutrient requirement. In nature, micronutrients are ubiquitous and probably do not usually limit growth. Micronutrients are normally a part of enzymes and cofactors, and they aid in the catalysis of reactions and maintenance of protein structure. For example, zinc (Zn2) is present at the active site of some enzymes but is also involved in the association of regulatory and catalytic subunits in E. coli aspartate carbamoyltransferase (see section 8.9). Manganese (Mn2) aids many enzymes catalyzing the transfer of phosphate groups. Molybdenum (Mo2) is required for nitrogen fixation, and cobalt (Co2) is a component of vitamin B12. Electron carriers and enzymes (pp. 157–64) 96 Chapter 5 Microbial Nutrition Besides the common macroelements and trace elements, microorganisms may have particular requirements that reflect the special nature of their morphology or environment. Diatoms (see figure 26.6c,d) need silicic acid (H4SiO4) to construct their beautiful cell walls of silica [(SiO2)n]. Although most bacteria do not require large amounts of sodium, many bacteria growing in saline lakes and oceans (see pp. 123, 461) depend on the presence of high concentrations of sodium ion (Na). Finally, it must be emphasized that microorganisms require a balanced mixture of nutrients. If an essential nutrient is in short supply, microbial growth will be limited regardless of the concentrations of other nutrients. 5.2 Requirements for Carbon, Hydrogen, and Oxygen The requirements for carbon, hydrogen, and oxygen often are satisfied together. Carbon is needed for the skeleton or backbone of all organic molecules, and molecules serving as carbon sources normally also contribute both oxygen and hydrogen atoms. They are the source of all three elements. Because these organic nutrients are almost always reduced and have electrons that they can donate to other molecules, they also can serve as energy sources. Indeed, the more reduced organic molecules are, the higher their energy content (e.g., lipids have a higher energy content than carbohydrates). This is because, as we shall see later, electron transfers release energy when the electrons move from reduced donors with more negative reduction potentials to oxidized electron acceptors with more positive potentials. Thus carbon sources frequently also serve as energy sources, although they don’t have to. Oxidation-reduction reactions and energy (pp. 157–59) One important carbon source that does not supply hydrogen or energy is carbon dioxide (CO2). This is because CO2 is oxidized and lacks hydrogen. Probably all microorganisms can fix CO2—that is, reduce it and incorporate it into organic molecules. However, by definition, only autotrophs can use CO2 as their sole or principal source of carbon. Many microorganisms are autotrophic, and most of these carry out photosynthesis and use light as their energy source. Some autotrophs oxidize inorganic molecules and derive energy from electron transfers. Photosynthetic carbon dioxide fixation (pp. 207–8) The reduction of CO2 is a very energy-expensive process. Thus many microorganisms cannot use CO2 as their sole carbon source but must rely on the presence of more reduced, complex molecules such as glucose for a supply of carbon. Organisms that use reduced, preformed organic molecules as carbon sources are heterotrophs (these preformed molecules normally come from other organisms). As mentioned previously, most heterotrophs use reduced organic compounds as sources of both carbon and energy. For example, the glycolytic pathway produces carbon skeletons for use in biosynthesis and also releases energy as ATP and NADH. The glycolytic pathway (pp. 176–77) A most remarkable nutritional characteristic of microorganisms is their extraordinary flexibility with respect to carbon sources. Laboratory experiments indicate that there is no natu-
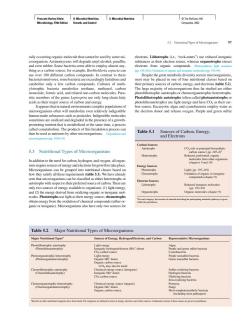
tena see electrons from organic compounds. use over 100 different carbon con unds.In contrast to thes pite the great metabolic diversity ria are exc ingy fastid ost may be placed in one of fou lotrophic m The pr ority of micro isms thus far studi ed are eithe nc- only long-chain fatty trophs)use light energy and have CO as thei Table 5.1 1010-14) 5.3 Nutritional Types of Microorganisms In addition to the peed for carbon.hvdroee .and oxygen,all or e(m1s-20 n长7GhTg o sources of energy available to organisms:(1)light energy e molecules ganic or inorganic).Microorganisms also have only two sources for Table 5.2 Major Nutritional Types of Microorganisms Major Nutritional Type Repre opaotoRtroop lectron (H/e)donor ce (inorganic) ulfur-oxidizing bacteri urce(organic
Prescott−Harley−Klein: Microbiology, Fifth Edition II. Microbial Nutrition, Growth, and Control 5. Microbial Nutrition © The McGraw−Hill Companies, 2002 rally occurring organic molecule that cannot be used by some microorganism. Actinomycetes will degrade amyl alcohol, paraffin, and even rubber. Some bacteria seem able to employ almost anything as a carbon source; for example, Burkholderia cepacia can use over 100 different carbon compounds. In contrast to these bacterial omnivores, some bacteria are exceedingly fastidious and catabolize only a few carbon compounds. Cultures of methylotrophic bacteria metabolize methane, methanol, carbon monoxide, formic acid, and related one-carbon molecules. Parasitic members of the genus Leptospira use only long-chain fatty acids as their major source of carbon and energy. It appears that in natural environments complex populations of microorganisms often will metabolize even relatively indigestible human-made substances such as pesticides. Indigestible molecules sometimes are oxidized and degraded in the presence of a growthpromoting nutrient that is metabolized at the same time, a process called cometabolism. The products of this breakdown process can then be used as nutrients by other microorganisms. Degradation and microorganisms (pp. 1010–14) 5.3 Nutritional Types of Microorganisms In addition to the need for carbon, hydrogen, and oxygen, all organisms require sources of energy and electrons for growth to take place. Microorganisms can be grouped into nutritional classes based on how they satisfy all these requirements (table 5.1). We have already seen that microorganisms can be classified as either heterotrophs or autotrophs with respect to their preferred source of carbon. There are only two sources of energy available to organisms: (1) light energy, and (2) the energy derived from oxidizing organic or inorganic molecules. Phototrophs use light as their energy source; chemotrophs obtain energy from the oxidation of chemical compounds (either organic or inorganic). Microorganisms also have only two sources for electrons. Lithotrophs (i.e., “rock-eaters”) use reduced inorganic substances as their electron source, whereas organotrophs extract electrons from organic compounds. Photosynthesis light reactions (pp. 195–201); Oxidation of organic and inorganic molecules (pp. 176–95) Despite the great metabolic diversity seen in microorganisms, most may be placed in one of four nutritional classes based on their primary sources of carbon, energy, and electrons (table 5.2). The large majority of microorganisms thus far studied are either photolithotrophic autotrophs or chemoorganotrophic heterotrophs. Photolithotrophic autotrophs (often called photoautotrophs or photolithoautotrophs) use light energy and have CO2 as their carbon source. Eucaryotic algae and cyanobacteria employ water as the electron donor and release oxygen. Purple and green sulfur 5.3 Nutritional Types of Microorganisms 97 Table 5.1 Sources of Carbon, Energy, and Electrons Carbon Sources Autotrophs CO2 sole or principal biosynthetic carbon source ( pp. 207–8) a Heterotrophs Reduced, preformed, organic molecules from other organisms (chapters 9 and 10) Energy Sources Phototrophs Light ( pp. 195–201) Chemotrophs Oxidation of organic or inorganic compounds (chapter 9) Electron Sources Lithotrophs Reduced inorganic molecules ( pp. 193–94) Organotrophs Organic molecules (chapter 9) a For each category, the location of material describing the participating metabolic pathways is given within the parentheses. Table 5.2 Major Nutritional Types of Microorganisms Major Nutritional Typesa Sources of Energy, Hydrogen/Electrons, and Carbon Representative Microorganisms Photolithotrophic autotrophy Light energy Algae (Photolithoautotrophy) Inorganic hydrogen/electron (H/e– ) donor Purple and green sulfur bacteria CO2 carbon source Cyanobacteria Photoorganotrophic heterotrophy Light energy Purple nonsulfur bacteria (Photoorganoheterotrophy) Organic H/e– donor Green nonsulfur bacteria Organic carbon source (CO2 may also be used) Chemolithotrophic autotrophy Chemical energy source (inorganic) Sulfur-oxidizing bacteria (Chemolithoautotrophy) Inorganic H/e– donor Hydrogen bacteria CO2 carbon source Nitrifying bacteria Iron-oxidizing bacteria Chemoorganotrophic heterotrophy Chemical energy source (organic) Protozoa (Chemoorganoheterotrophy) Organic H/e– donor Fungi Organic carbon source Most nonphotosynthetic bacteria (including most pathogens) a Bacteria in other nutritional categories have been found. The categories are defined in terms of energy, electron, and carbon sources. Condensed versions of these names are given in parentheses

5.Microbial Nutrition Chapter 5 Microbial Nutrition ena ns from in 5orrogen,Phmporu chemo. rotrophs,chemoorganoneterotr ohs,or ev Togrow.a microorganism must be able to large quanti- all th lly all croorganisms usually employ inorganic sources as well.Bioch The have fewermi sms Nitrogen is needed for the synthesis of amino acids purne some ca ohydrates and lipids.enzyme co donor The photoorgan het in amino acids.and amn monia ofen is directly incorporate nic o rogen,or h energy and elec eria many cyanobacteria and the symbiotic bacte ospheric nitrogen usin c the the y e monia to nitrate or sulfur to sulfate)that continally occur in the nents. Almost all microorgan ms use inorg pho phosphorus s cies ually belongs ino ents.Phosphate uptake by coli ha ty and alter their meta sively n can use lic nmen 0n220 example.many purp the enzyr which then is the plasma membrane example is provided by bac cria such as Beg the outer membrane by the use of a porin protein channel.One ccause they probably is due to the Pit system.When phosphate ons are low,th This sort of flexibility seems co mplex and confusing.vet it give mpecycatrnieadaniaeifcmioamendcomdioas for phosphate:it isan ABC transporter (see pp.101)anduse P amino acids cysteir and methionine,some carbohydrates,bi- tion 10.4):a few require a reduced form of sulfur such as cysteine. otrophand he roph tremsotcergyndcecton d o 5.5 Growth Factors Microorganisms often grow and reproduce when minerals and
Prescott−Harley−Klein: Microbiology, Fifth Edition II. Microbial Nutrition, Growth, and Control 5. Microbial Nutrition © The McGraw−Hill Companies, 2002 bacteria cannot oxidize water but extract electrons from inorganic donors like hydrogen, hydrogen sulfide, and elemental sulfur. Chemoorganotrophic heterotrophs (often called chemoheterotrophs, chemoorganoheterotrophs, or even heterotrophs) use organic compounds as sources of energy, hydrogen, electrons, and carbon. Frequently the same organic nutrient will satisfy all these requirements. It should be noted that essentially all pathogenic microorganisms are chemoheterotrophs. The other two nutritional classes have fewer microorganisms but often are very important ecologically. Some purple and green bacteria are photosynthetic and use organic matter as their electron donor and carbon source. These photoorganotrophic heterotrophs (photoorganoheterotrophs) are common inhabitants of polluted lakes and streams. Some of these bacteria also can grow as photoautotrophs with molecular hydrogen as an electron donor. The fourth group, the chemolithotrophic autotrophs (chemolithoautotrophs), oxidizes reduced inorganic compounds such as iron, nitrogen, or sulfur molecules to derive both energy and electrons for biosynthesis. Carbon dioxide is the carbon source. A few chemolithotrophs can derive their carbon from organic sources and thus are heterotrophic. Chemolithotrophs contribute greatly to the chemical transformations of elements (e.g., the conversion of ammonia to nitrate or sulfur to sulfate) that continually occur in the ecosystem. Photosynthetic and chemolithotrophic bacteria (sections 21.3, 22.1, and 22.3) Although a particular species usually belongs in only one of the four nutritional classes, some show great metabolic flexibility and alter their metabolic patterns in response to environmental changes. For example, many purple nonsulfur bacteria (see section 22.1) act as photoorganotrophic heterotrophs in the absence of oxygen but oxidize organic molecules and function chemotrophically at normal oxygen levels. When oxygen is low, photosynthesis and oxidative metabolism may function simultaneously. Another example is provided by bacteria such as Beggiatoa (see p. 501) that rely on inorganic energy sources and organic (or sometimes CO2) carbon sources. These microbes are sometimes called mixotrophic because they combine chemolithoautotrophic and heterotrophic metabolic processes. This sort of flexibility seems complex and confusing, yet it gives its possessor a definite advantage if environmental conditions frequently change. 1. What are nutrients, and on what basis are they divided into macroelements and micronutrients or trace elements? Describe some ways in which macroelements and micronutrients are used by an organism. 2. Define autotroph and heterotroph. 3. Discuss the ways in which microorganisms are classified based on their requirements for energy and electrons. 4. Describe the nutritional requirements of the four major nutritional groups and give some microbial examples of each. What is a mixotroph? 5.4 Requirements for Nitrogen, Phosphorus, and Sulfur To grow, a microorganism must be able to incorporate large quantities of nitrogen, phosphorus, and sulfur. Although these elements may be acquired from the same nutrients that supply carbon, microorganisms usually employ inorganic sources as well. Biochemical mechanisms for the incorporation of nitrogen, phosphorus, and sulfur (pp. 210–14) Nitrogen is needed for the synthesis of amino acids, purines, pyrimidines, some carbohydrates and lipids, enzyme cofactors, and other substances. Many microorganisms can use the nitrogen in amino acids, and ammonia often is directly incorporated through the action of such enzymes as glutamate dehydrogenase or glutamine synthetase and glutamate synthase (see section 10.4). Most phototrophs and many nonphotosynthetic microorganisms reduce nitrate to ammonia and incorporate the ammonia in assimilatory nitrate reduction (see pp. 210–11). A variety of bacteria (e.g., many cyanobacteria and the symbiotic bacterium Rhizobium) can reduce and assimilate atmospheric nitrogen using the nitrogenase system (see section 10.4). Phosphorus is present in nucleic acids, phospholipids, nucleotides like ATP, several cofactors, some proteins, and other cell components. Almost all microorganisms use inorganic phosphate as their phosphorus source and incorporate it directly. Low phosphate levels actually limit microbial growth in many aquatic environments. Phosphate uptake by E. coli has been intensively studied. This bacterium can use both organic and inorganic phosphate. Some organophosphates such as hexose 6-phosphates can be taken up directly by transport proteins. Other organophosphates are often hydrolyzed in the periplasm by the enzyme alkaline phosphatase to produce inorganic phosphate, which then is transported across the plasma membrane. When inorganic phosphate is outside the bacterium, it crosses the outer membrane by the use of a porin protein channel. One of two transport systems subsequently moves the phosphate across the plasma membrane. At high phosphate concentrations, transport probably is due to the Pit system. When phosphate concentrations are low, the PST, (phosphate-specific transport) system is more important. The PST system has higher affinity for phosphate; it is an ABC transporter (see pp. 101–2) and uses a periplasmic binding protein. Sulfur is needed for the synthesis of substances like the amino acids cysteine and methionine, some carbohydrates, biotin, and thiamine. Most microorganisms use sulfate as a source of sulfur and reduce it by assimilatory sulfate reduction (see section 10.4); a few require a reduced form of sulfur such as cysteine. 5.5 Growth Factors Microorganisms often grow and reproduce when minerals and sources of energy, carbon, nitrogen, phosphorus, and sulfur are supplied. These organisms have the enzymes and pathways nec- 98 Chapter 5 Microbial Nutrition

8e Table 5.3 Functions of Some Common Vitamins in Microorganisms Vitamin Functions Examples of Microorganisms Requiring Vitamin' B Folic acid is (B Lipoic acid Transfer of acyl groups (P spp(P (B) Niacin (ncn) neaaaadNbR-terydkaem (B) Riboflavin (B.) Nryero (B) is (B) ctera (B).fanp (algae (A)and pe ssary to synthesize all cell comnonents required for their well- used in microbiological assays of most vitamins and amino acids componentsex cept the wth factor to beass aved.A differen amount of grow factor is added to e ach vessel.The standard re are thre sses th Ta th resulting i ynthesis purines and pyrimidinesfor mins are mo tity of t vi large au le and a '0r many microorganisms nakes the microor that synth For example,spec
Prescott−Harley−Klein: Microbiology, Fifth Edition II. Microbial Nutrition, Growth, and Control 5. Microbial Nutrition © The McGraw−Hill Companies, 2002 essary to synthesize all cell components required for their wellbeing. Many microorganisms, on the other hand, lack one or more essential enzymes. Therefore they cannot manufacture all indispensable constituents but must obtain them or their precursors from the environment. Organic compounds required because they are essential cell components or precursors of such components and cannot be synthesized by the organism are called growth factors. There are three major classes of growth factors: (1) amino acids, (2) purines and pyrimidines, and (3) vitamins. Amino acids are needed for protein synthesis, purines and pyrimidines for nucleic acid synthesis. Vitamins are small organic molecules that usually make up all or part of enzyme cofactors (see section 8.6), and only very small amounts sustain growth. The functions of selected vitamins, and examples of microorganisms requiring them, are given in table 5.3. Some microorganisms require many vitamins; for example, Enterococcus faecalis needs eight different vitamins for growth. Other growth factors are also seen; heme (from hemoglobin or cytochromes) is required by Haemophilus influenzae, and some mycoplasmas need cholesterol. Knowledge of the specific growth factor requirements of many microorganisms makes possible quantitative growthresponse assays for a variety of substances. For example, species from the bacterial genera Lactobacillus and Streptococcus can be used in microbiological assays of most vitamins and amino acids. The appropriate bacterium is grown in a series of culture vessels, each containing medium with an excess amount of all required components except the growth factor to be assayed. A different amount of growth factor is added to each vessel. The standard curve is prepared by plotting the growth factor quantity or concentration against the total extent of bacterial growth. Ideally the amount of growth resulting is directly proportional to the quantity of growth factor present; if the growth factor concentration doubles, the final extent of bacterial growth doubles. The quantity of the growth factor in a test sample is determined by comparing the extent of growth caused by the unknown sample with that resulting from the standards. Microbiological assays are specific, sensitive, and simple. They still are used in the assay of substances like vitamin B12 and biotin, despite advances in chemical assay techniques. The observation that many microorganisms can synthesize large quantities of vitamins has led to their use in industry. Several water-soluble and fat-soluble vitamins are produced partly or completely using industrial fermentations. Good examples of such vitamins and the microorganisms that synthesize them are riboflavin (Clostridium, Candida, Ashbya, Eremothecium), coenzyme A (Brevibacterium), vitamin B12 (Streptomyces, Propionibacterium, 5.5 Growth Factors 99 Table 5.3 Functions of Some Common Vitamins in Microorganisms Vitamin Functions Examples of Microorganisms Requiring Vitamina Biotin Carboxylation (CO2 fixation) Leuconostoc mesenteroides (B) One-carbon metabolism Saccharomyces cerevisiae (F) Ochromonas malhamensis (A) Acanthamoeba castellanii (P) Cyanocobalamin (B12) Molecular rearrangements Lactobacillus spp. (B) One-carbon metabolism—carries methyl groups Euglena gracilis (A) Diatoms and many other algae (A) Acanthamoeba castellanii (P) Folic acid One-carbon metabolism Enterococcus faecalis (B) Tetrahymena pyriformis (P) Lipoic acid Transfer of acyl groups Lactobacillus casei (B) Tetrahymena spp. (P) Pantothenic acid Precursor of coenzyme A—carries acyl groups Proteus morganii (B) (pyruvate oxidation, fatty acid metabolism) Hanseniaspora spp. (F) Paramecium spp. (P) Pyridoxine (B6) Amino acid metabolism (e.g., transamination) Lactobacillus spp. (B) Tetrahymena pyriformis (P) Niacin (nicotinic acid) Precursor of NAD and NADP—carry electrons Brucella abortus, Haemophilus influenzae (B) and hydrogen atoms Blastocladia pringsheimii (F) Crithidia fasciculata (P) Riboflavin (B2) Precursor of FAD and FMN—carry electrons Caulobacter vibrioides (B) or hydrogen atoms Dictyostelium spp. (F) Tetrahymena pyriformis (P) Thiamine (B1) Aldehyde group transfer Bacillus anthracis (B) (pyruvate decarboxylation, α-keto acid oxidation) Phycomyces blakesleeanus (F) Ochromonas malhamensis (A) Colpidium campylum (P) a The representative microorganisms are members of the following groups: bacteria (B), fungi (F), algae (A), and protozoa (P)

玉Microbial Nutrition 100 Pseudon ganisms that can produce large quantities of other vitamins. substance in a sample? 5.6 Uptake of Nutrients by the Cell t 5.1 Facitated dependence of acriliaicdicetorplt bove a s free pas ncIn view of the o The most important of these are facilitated diffusion.active trans ure5.1).Note that the diffusion rate levels offor reachesaplateau gradient value ecause the carner is saturate ssible.The res emyme-subsrae cve e s different fro Facilitated Diffusion substance to be erol,can cross the plasma mem no metabolic energy input is required.If the concentration gradi ound or by moving it to another membranous compartment uptake by p ve di cucaryotes. ese camiers are ated t the rate of uptake decreases as more nutrient i ngtothe MIP family of proteins.The two most widespread MIP sed imi diately.Very small mo an ndpolr substance do notcromm Although much work has been done on the mechanism of fa cell interio.The carrier would change sion es with the con shapc and be ready to pick up another mole he ne ve diffusion (fi sponse to its co centration gradient.Remember that the mecha
Prescott−Harley−Klein: Microbiology, Fifth Edition II. Microbial Nutrition, Growth, and Control 5. Microbial Nutrition © The McGraw−Hill Companies, 2002 Pseudomonas), vitamin C (Gluconobacter, Erwinia, Corynebacterium), -carotene (Dunaliella), and vitamin D (Saccharomyces). Current research focuses on improving yields and finding microorganisms that can produce large quantities of other vitamins. 1. Briefly summarize the ways in which microorganisms obtain nitrogen, phosphorus, and sulfur from their environment. 2. What are growth factors? What are vitamins? How can microorganisms be used to determine the quantity of a specific substance in a sample? 5.6 Uptake of Nutrients by the Cell The first step in nutrient use is uptake of the required nutrients by the microbial cell. Uptake mechanisms must be specific—that is, the necessary substances, and not others, must be acquired. It does a cell no good to take in a substance that it cannot use. Since microorganisms often live in nutrient-poor habitats, they must be able to transport nutrients from dilute solutions into the cell against a concentration gradient. Finally, nutrient molecules must pass through a selectively permeable plasma membrane that will not permit the free passage of most substances. In view of the enormous variety of nutrients and the complexity of the task, it is not surprising that microorganisms make use of several different transport mechanisms. The most important of these are facilitated diffusion, active transport, and group translocation. Eucaryotic microorganisms do not appear to employ group translocation but take up nutrients by the process of endocytosis (see section 4.5). Plasma membrane structure and properties (pp. 46–48) Facilitated Diffusion A few substances, such as glycerol, can cross the plasma membrane by passive diffusion. Passive diffusion, often simply called diffusion, is the process in which molecules move from a region of higher concentration to one of lower concentration because of random thermal agitation. The rate of passive diffusion is dependent on the size of the concentration gradient between a cell’s exterior and its interior (figure 5.1). A fairly large concentration gradient is required for adequate nutrient uptake by passive diffusion (i.e., the external nutrient concentration must be high), and the rate of uptake decreases as more nutrient is acquired unless it is used immediately. Very small molecules such as H2O, O2, and CO2 often move across membranes by passive diffusion. Larger molecules, ions, and polar substances do not cross membranes by passive or simple diffusion. The rate of diffusion across selectively permeable membranes is greatly increased by using carrier proteins, sometimes called permeases, which are embedded in the plasma membrane. Because a carrier aids the diffusion process, it is called facilitated diffusion. The rate of facilitated diffusion increases with the concentration gradient much more rapidly and at lower concentrations of the diffusing molecule than that of passive diffusion (figure 5.1). Note that the diffusion rate levels off or reaches a plateau above a specific gradient value because the carrier is saturated— that is, the carrier protein is binding and transporting as many solute molecules as possible. The resulting curve resembles an enzyme-substrate curve (see section 8.6) and is different from the linear response seen with passive diffusion. Carrier proteins also resemble enzymes in their specificity for the substance to be transported; each carrier is selective and will transport only closely related solutes. Although a carrier protein is involved, facilitated diffusion is truly diffusion. A concentration gradient spanning the membrane drives the movement of molecules, and no metabolic energy input is required. If the concentration gradient disappears, net inward movement ceases. The gradient can be maintained by transforming the transported nutrient to another compound or by moving it to another membranous compartment in eucaryotes. Interestingly, some of these carriers are related to the major intrinsic protein of mammalian eye lenses and thus belong to the MIP family of proteins. The two most widespread MIP channels in bacteria are aquaporins that transport water and glycerol facilitators, which aid glycerol diffusion. Although much work has been done on the mechanism of facilitated diffusion, the process is not yet understood completely. It appears that the carrier protein complex spans the membrane (figure 5.2). After the solute molecule binds to the outside, the carrier may change conformation and release the molecule on the cell interior. The carrier would subsequently change back to its original shape and be ready to pick up another molecule. The net effect is that a lipid-insoluble molecule can enter the cell in response to its concentration gradient. Remember that the mecha- 100 Chapter 5 Microbial Nutrition Concentration gradient Rate of transport Passive diffusion Facilitated diffusion Figure 5.1 Passive and Facilitated Diffusion. The dependence of diffusion rate on the size of the solute’s concentration gradient. Note the saturation effect or plateau above a specific gradient value when a facilitated diffusion carrier is operating. This saturation effect is seen whenever a carrier protein is involved in transport.

5.6 Uptake of Nutrients by the Cell 101 2 ATP ADP ABC a er complex..(②)Tm ngpwoteinaaC will move outward.Because the cell metabolizes nutrients upor ilar solute molecules can compete for the same carrier protein in does not seem to be im yotes because nutrient con trations(佰gure5..Nevertheles. fomfaciitatoddisionniusecofmctaboicsnegyand onas,Bacillus,and many other ba energy prod faci m ATP-binding transporters(ABCtransporters)are active in bac Active Transport two nucltid-inding cannot take up solutes that are al- bran and the nucleouide-binding domains bind and h eady more con rces,and,too rish.thev ram-r amma ese nu s.Thus xternal face o transpo he (s).bind the molecule to situations are active transport and group Active transportis the transport of solute molecules gars (arab maltose,galactose,ribose)and amino acids ort in gm by th volves protein carrier activity,it resembles facilitated diffusion in
Prescott−Harley−Klein: Microbiology, Fifth Edition II. Microbial Nutrition, Growth, and Control 5. Microbial Nutrition © The McGraw−Hill Companies, 2002 nism is driven by concentration gradients and therefore is reversible. If the solute’s concentration is greater inside the cell, it will move outward. Because the cell metabolizes nutrients upon entry, influx is favored. Facilitated diffusion does not seem to be important in procaryotes because nutrient concentrations often are lower outside the cell so that facilitated diffusion cannot be used in uptake. Glycerol is transported by facilitated diffusion in E. coli, Salmonella typhimurium, Pseudomonas, Bacillus, and many other bacteria. The process is much more prominent in eucaryotic cells where it is used to transport a variety of sugars and amino acids. Active Transport Although facilitated diffusion carriers can efficiently move molecules to the interior when the solute concentration is higher on the outside of the cell, they cannot take up solutes that are already more concentrated within the cell (i.e., against a concentration gradient). Microorganisms often live in habitats characterized by very dilute nutrient sources, and, to flourish, they must be able to transport and concentrate these nutrients. Thus facilitated diffusion mechanisms are not always adequate, and other approaches must be used. The two most important transport processes in such situations are active transport and group translocation, both energy-dependent processes. Active transport is the transport of solute molecules to higher concentrations, or against a concentration gradient, with the use of metabolic energy input. Because active transport involves protein carrier activity, it resembles facilitated diffusion in some ways. The carrier proteins or permeases bind particular solutes with great specificity for the molecules transported. Similar solute molecules can compete for the same carrier protein in both facilitated diffusion and active transport. Active transport is also characterized by the carrier saturation effect at high solute concentrations (figure 5.1). Nevertheless, active transport differs from facilitated diffusion in its use of metabolic energy and in its ability to concentrate substances. Metabolic inhibitors that block energy production will inhibit active transport but will not affect facilitated diffusion (at least for a short time). Binding protein transport systems or ATP-binding cassette transporters (ABC transporters) are active in bacteria, archaea, and eucaryotes. Usually these transporters consist of two hydrophobic membrane-spanning domains associated on their cytoplasmic surfaces with two nucleotide-binding domains (figure 5.3). The membrane-spanning domains form a pore in the membrane and the nucleotide-binding domains bind and hydrolyze ATP to drive uptake. ABC transporters employ special substrate binding proteins, which are located in the periplasmic space of gram-negative bacteria (see figure 3.23) or are attached to membrane lipids on the external face of the gram-positive plasma membrane. These binding proteins, which also may participate in chemotaxis (see pp. 66–68), bind the molecule to be transported and then interact with the membrane transport proteins to move the solute molecule inside the cell. E. coli transports a variety of sugars (arabinose, maltose, galactose, ribose) and amino acids (glutamate, histidine, leucine) by this mechanism. Substances entering gram-negative bacteria must pass through the outer membrane before ABC transporters and other 5.6 Uptake of Nutrients by the Cell 101 Inside the cell Outside the cell Plasma membrane Figure 5.2 A Model of Facilitated Diffusion. The membrane carrier can change conformation after binding an external molecule and subsequently release the molecule on the cell interior. It then returns to the outward oriented position and is ready to bind another solute molecule. Because there is no energy input, molecules will continue to enter only as long as their concentration is greater on the outside. ATP 1 2 Solutebinding protein Transporter Nucleotidebinding domain Cytoplasmic matrix Periplasm ADP + Pi ATP ADP + Pi Figure 5.3 ABC Transporter Function. (1) The solute binding protein binds the substrate to be transported and approaches the ABC transporter complex. (2) The solute binding protein attaches to the transporter and releases the substrate, which is moved across the membrane with the aid of ATP hydrolysis. See text for details
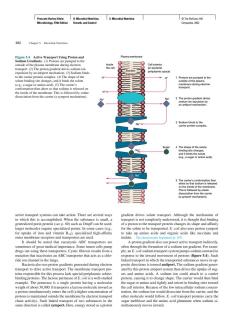
5.Microbial Nutrition 02 Chapter 5 Microbial Nutrition of h d to th active transport systems can take action.There are several v ient drives solute transport.Although the mechanism of in which this.When the substance s smll. for the solute to be transported e coli also uses proton sympor ake up amino acids and organic acids like succinate and It should be noted that eucaryotie ABC transpo rters are A proton gradient also can power active transport indirectly s pump aEoghteomaionofasodinmiomgnd ent.For cxar ates an ABC transporter that acts as a chlo c to the inward ms(figure5.4.Such transport to drive active transport.The membrane transport pro ted by this proton antipor system then drives the uptake of sug oton simultan protons is maintained e the membr other mol multaneously moves inward
Prescott−Harley−Klein: Microbiology, Fifth Edition II. Microbial Nutrition, Growth, and Control 5. Microbial Nutrition © The McGraw−Hill Companies, 2002 active transport systems can take action. There are several ways in which this is accomplished. When the substance is small, a generalized porin protein (see p. 60) such as OmpF can be used; larger molecules require specialized porins. In some cases (e.g., for uptake of iron and vitamin B12), specialized high-affinity outer membrane receptors and transporters are used. It should be noted that eucaryotic ABC transporters are sometimes of great medical importance. Some tumor cells pump drugs out using these transporters. Cystic fibrosis results from a mutation that inactivates an ABC transporter that acts as a chloride ion channel in the lungs. Bacteria also use proton gradients generated during electron transport to drive active transport. The membrane transport proteins responsible for this process lack special periplasmic solutebinding proteins. The lactose permease of E. coli is a well-studied example. The permease is a single protein having a molecular weight of about 30,000. It transports a lactose molecule inward as a proton simultaneously enters the cell (a higher concentration of protons is maintained outside the membrane by electron transport chain activity). Such linked transport of two substances in the same direction is called symport. Here, energy stored as a proton gradient drives solute transport. Although the mechanism of transport is not completely understood, it is thought that binding of a proton to the transport protein changes its shape and affinity for the solute to be transported. E. coli also uses proton symport to take up amino acids and organic acids like succinate and malate. The chemiosmotic hypothesis (p. 187) A proton gradient also can power active transport indirectly, often through the formation of a sodium ion gradient. For example, an E. coli sodium transport system pumps sodium outward in response to the inward movement of protons (figure 5.4). Such linked transport in which the transported substances move in opposite directions is termed antiport. The sodium gradient generated by this proton antiport system then drives the uptake of sugars and amino acids. A sodium ion could attach to a carrier protein, causing it to change shape. The carrier would then bind the sugar or amino acid tightly and orient its binding sites toward the cell interior. Because of the low intracellular sodium concentration, the sodium ion would dissociate from the carrier, and the other molecule would follow. E. coli transport proteins carry the sugar melibiose and the amino acid glutamate when sodium simultaneously moves inward. 102 Chapter 5 Microbial Nutrition Cell exterior (or bacterial periplasmic space) Inside the cell Electron transport 1 2 Sugar 4 3 5 Protons are pumped to the outside of the plasma membrane during electron transport. The proton gradient drives sodium ion expulsion by an antiport mechanism. The shape of the solute binding site changes, and it binds the solute (e.g., a sugar or amino acid). Sodium binds to the carrier protein complex. The carrier’s conformation then alters so that sodium is released on the inside of the membrane. This is followed by solute dissociation from the carrier (a symport mechanism). Plasma membrane H H+ + H+ Na+ Na+ Na+ Na+ Na+ Figure 5.4 Active Transport Using Proton and Sodium Gradients. (1) Protons are pumped to the outside of the plasma membrane during electron transport. (2) The proton gradient drives sodium ion expulsion by an antiport mechanism. (3) Sodium binds to the carrier protein complex. (4) The shape of the solute binding site changes, and it binds the solute (e.g., a sugar or amino acid). (5) The carrier’s conformation then alters so that sodium is released on the inside of the membrane. This is followed by solute dissociation from the carrier (a symport mechanism)

5.6 Uptake of Nutrients by the Cell 103 X 55G hed to EllB in th ElB in the s es from Sodium s alco ic an i )The bolic Often a microorganism has more than one tran sport systen the phosphate donor. PEP+sgar((o)→p ruvate+sugar-P(inside) ems each for the amino acids glutamate and leucine and twe tems for the same substance.the sy prop heat-stabe cntnmctreandoi composed of its poss variable environment a hydr the me EPto GroupTranslocation d as it is camed acr whereas nd HPr fied stype of nergy-dependenttrao because meta ferase system,aerobic bacteria seem to lack PTSs.Members of the
Prescott−Harley−Klein: Microbiology, Fifth Edition II. Microbial Nutrition, Growth, and Control 5. Microbial Nutrition © The McGraw−Hill Companies, 2002 Sodium symport or cotransport also is an important process in eucaryotic cells where it is used in sugar and amino acid uptake. ATP, rather than proton motive force, usually drives sodium transport in eucaryotic cells. Often a microorganism has more than one transport system for each nutrient, as can be seen with E. coli. This bacterium has at least five transport systems for the sugar galactose, three systems each for the amino acids glutamate and leucine, and two potassium transport complexes. When there are several transport systems for the same substance, the systems differ in such properties as their energy source, their affinity for the solute transported, and the nature of their regulation. Presumably this diversity gives its possessor an added competitive advantage in a variable environment. Group Translocation In active transport, solute molecules move across a membrane without modification. Many procaryotes also take up molecules by group translocation, a process in which a molecule is transported into the cell while being chemically altered (this can be classified as a type of energy-dependent transport because metabolic energy is used). The best-known group translocation system is the phosphoenolpyruvate: sugar phosphotransferase system (PTS). It transports a variety of sugars into procaryotic cells while phosphorylating them using phosphoenolpyruvate (PEP) as the phosphate donor. PEP sugar (outside) → pyruvate sugar P (inside) The PTS is quite complex. In E. coli and Salmonella typhimurium, it consists of two enzymes and a low molecular weight heat-stable protein (HPr). HPr and enzyme I (EI) are cytoplasmic. Enzyme II (EII) is more variable in structure and often composed of three subunits or domains. EIIA (formerly called EIII) is cytoplasmic and soluble. EIIB also is hydrophilic but frequently is attached to EIIC, a hydrophobic protein that is embedded in the membrane. A high-energy phosphate is transferred from PEP to enzyme II with the aid of enzyme I and HPr (figure 5.5). Then, a sugar molecule is phosphorylated as it is carried across the membrane by enzyme II. Enzyme II transports only specific sugars and varies with PTS, whereas enzyme I and HPr are common to all PTSs. PTSs are widely distributed in procaryotes. Except for some species of Bacillusthat have both glycolysis and the phosphotransferase system, aerobic bacteria seem to lack PTSs. Members of the 5.6 Uptake of Nutrients by the Cell 103 Mannitol-1-P IIA IIB P P P P ~ HPr~ P Glucose-6-P HPr Cytoplasmic matrix Periplasm Glucose Mannitol IIA IIC EI EI~ P PEP Pyruvate IIB IIC ~ ~ ~ Figure 5.5 Group Translocation: Bacterial PTS Transport. Two examples of the phosphoenolpyruvate: sugar phosphotransferase system (PTS) are illustrated. The following components are involved in the system: phosphoenolpyruvate (PEP), enzyme I (EI), the low molecular weight heat-stable protein (HPr), and enzyme II (EII). The high-energy phosphate is transferred from HPr to the soluble EIIA. EIIA is attached to EIIB in the mannitol transport system and is separate from EIIB in the glucose system. In either case the phosphate moves from EIIA to EIIB, and then is transferred to the sugar during transport through the membrane. Other relationships between the EII components are possible. For example, IIA and IIB may form a soluble protein separate from the membrane complex; the phosphate still moves from IIA to IIB and then to the membrane domain(s).

5.Microbial Nutrition 04 Chapter 5 Microbial Nutritio CH, when little iron is ava n the me ore complex ha d th N-C CH: CHC%MH-CH一CO membrane of the cell envelope:when the iron ach bactin-iron co nore tha ensure an adequate supply. 5.7 Culture Media Figure 5.0 Sidero .(b) mplex nism requires for gro th enera Escherichia.Salmonella.Staphvlococcus.and other facul tatively anacrobic bacteria (see p./27)have phosphotransferas arbon nitrogen phe phorus.sulfur.and various minerals.the so greatly.Knowledge of a microorganism's as chemoreceptors for chemotaxis. Iron Uptake dings.Frequently a medium is used to select and gro Almost all micr many nzymes.rn composition. Synthetic or Defined Media ito the cell.These atively simple media containing Cs a carbon soure (ofter a nitrogen source,sulfate,phosphate,and a variety of minerals
Prescott−Harley−Klein: Microbiology, Fifth Edition II. Microbial Nutrition, Growth, and Control 5. Microbial Nutrition © The McGraw−Hill Companies, 2002 genera Escherichia, Salmonella, Staphylococcus, and other facultatively anaerobic bacteria (see p. 127 ) have phosphotransferase systems; some obligately anaerobic bacteria (e.g., Clostridium) also have PTSs. Many carbohydrates are transported by these systems. E. coli takes up glucose, fructose, mannitol, sucrose, Nacetylglucosamine, cellobiose, and other carbohydrates by group translocation. Besides their role in transport, PTS proteins can act as chemoreceptors for chemotaxis. Iron Uptake Almost all microorganisms require iron for use in cytochromes and many enzymes. Iron uptake is made difficult by the extreme insolubility of ferric iron (Fe3) and its derivatives, which leaves little free iron available for transport. Many bacteria and fungi have overcome this difficulty by secreting siderophores [Greek for iron bearers]. Siderophores are low molecular weight molecules that are able to complex with ferric iron and supply it to the cell. These iron-transport molecules are normally either hydroxamates or phenolatescatecholates. Ferrichrome is a hydroxamate produced by many fungi; enterobactin is the catecholate formed by E. coli (figure 5.6a,b). It appears that three siderophore groups complex with iron orbitals to form a six-coordinate, octahedral complex (figure 5.6c). Microorganisms secrete siderophores when little iron is available in the medium. Once the iron-siderophore complex has reached the cell surface, it binds to a siderophore-receptor protein. Then the iron is either released to enter the cell directly or the whole iron-siderophore complex is transported inside by an ABC transporter. In E. coli the siderophore receptor is in the outer membrane of the cell envelope; when the iron reaches the periplasmic space, it moves through the plasma membrane with the aid of the transporter. After the iron has entered the cell, it is reduced to the ferrous form (Fe2). Iron is so crucial to microorganisms that they may use more than one route of iron uptake to ensure an adequate supply. 1. Describe facilitated diffusion, active transport, and group translocation in terms of their distinctive characteristics and mechanisms. 2. How do binding protein transport systems and membrane-bound transport systems differ with respect to energy sources? What are symport and antiport processes? 3. How are siderophores involved in iron transport? 5.7 Culture Media Much of the study of microbiology depends on the ability to grow and maintain microorganisms in the laboratory, and this is possible only if suitable culture media are available. A culture medium is a solid or liquid preparation used to grow, transport, and store microorganisms. To be effective, the medium must contain all the nutrients the microorganism requires for growth. Specialized media are essential in the isolation and identification of microorganisms, the testing of antibiotic sensitivities, water and food analysis, industrial microbiology, and other activities. Although all microorganisms need sources of energy, carbon, nitrogen, phosphorus, sulfur, and various minerals, the precise composition of a satisfactory medium will depend on the species one is trying to cultivate because nutritional requirements vary so greatly. Knowledge of a microorganism’s normal habitat often is useful in selecting an appropriate culture medium because its nutrient requirements reflect its natural surroundings. Frequently a medium is used to select and grow specific microorganisms or to help identify a particular species. In such cases the function of the medium also will determine its composition. Synthetic or Defined Media Some microorganisms, particularly photolithotrophic autotrophs such as cyanobacteria and eucaryotic algae, can be grown on relatively simple media containing CO2 as a carbon source (often added as sodium carbonate or bicarbonate), nitrate or ammonia as a nitrogen source, sulfate, phosphate, and a variety of minerals 104 Chapter 5 Microbial Nutrition NH (CO CH CH2 O)3 NH O C O O O O O C O N H HN O O C CH3 C N O O (CH2)3 Fe3+ O O Fe3+ (NH CH2 CO)3 (NH CH CO)3 C O Iron Enterobactin–iron complex Ferrichrome Enterobactin Figure 5.6 Siderophore Ferric Iron Complexes. (a) Ferrichrome is a cyclic hydroxamate [—CO—N(O)—] molecule formed by many fungi. (b) E. coli produces the cyclic catecholate derivative, enterobactin. (c) Ferric iron probably complexes with three siderophore groups to form a six-coordinate, octahedral complex as shown in this illustration of the enterobactin-iron complex. (a) (b) (c)
按次数下载不扣除下载券;
注册用户24小时内重复下载只扣除一次;
顺序:VIP每日次数-->可用次数-->下载券;
- 《环境微生物学与实验》课程教学资源(文献资料)Eucaryotic Cell Structure and Function.pdf
- 《环境微生物学与实验》课程教学资源(文献资料)Microbial Growth.pdf
- 《环境微生物学与实验》课程教学资源(文献资料)Control of Microorganisms by Physical and Chemical Agents.pdf
- 《环境微生物学与实验》课程教学资源(文献资料)The Viruses - Bacteriophages.pdf
- 《环境微生物学与实验》课程教学资源(文献资料)微生物实验室手册(外文版)A Digital Manual for Medical Microbiology Laboratory..pdf
- 《环境微生物学与实验》课程教学资源(文献资料)认识微生物.pdf
- 南京农业大学:《遗传学实验》课程教学大纲.pdf
- 南京农业大学:《动植物野外实习》课程教学大纲.pdf
- 南京农业大学:《细胞遗传学(双语)》课程教学大纲.pdf
- 南京农业大学:《前沿生命科学的奥秘》课程教学大纲.pdf
- 南京农业大学:《分子生物学》课程教学大纲.pdf
- 南京农业大学:《进化生态学》课程教学大纲.pdf
- 南京农业大学:《医学分子生物学》课程教学大纲.pdf
- 南京农业大学:《微生物资源与分类学》课程教学大纲.pdf
- 南京农业大学:《基础微生物学》课程教学大纲.pdf
- 南京农业大学:《生命科学导论》课程教学大纲.pdf
- 南京农业大学:《细胞信号转导》课程教学大纲.pdf
- 南京农业大学:《普通真菌学》课程教学大纲.pdf
- 南京农业大学:《动植物野外实习》课程教学大纲.pdf
- 南京农业大学:《分子生物学》课程教学大纲.pdf
- 《环境微生物学与实验》课程教学资源(文献资料)The History and Scope of Microbiology.pdf
- 《环境微生物学与实验》课程教学资源(文献资料)The Study of Microbial Structure - Microscopy and Specimen Preparation.pdf
- 同济大学:《环境工程微生物学》课程教学资源(试卷习题)考试试卷及答案.pdf
- 同济大学:《细胞的生物化学》课程教学资源(教学大纲)英文细胞生化理论留学生教学大纲 Syllabus for cellular biochemistry.docx
- 同济大学:《细胞的生物化学》课程教学资源(教学大纲)中文细胞生化理论留学生教学大纲.docx
- 同济大学:《细胞的生物化学》课程教学资源(试卷习题)2012-2013 Final term examination for Cellular Biochemistry(无答案).pdf
- 同济大学:《细胞的生物化学》课程电子教案(PPT课件)Genetic information transfer(RNA transcription 转录)RNA biosynthesis.pptx
- 同济大学:《细胞的生物化学》课程电子教案(PPT课件)Genetic information transfer——Protein Biosynthesis Translation(翻译).pptx
- 同济大学:《细胞的生物化学》课程电子教案(课件讲稿)细胞生物学(理论).pdf
- 同济大学:《细胞的生物化学》课程电子教案(课件讲稿)蛋白质 Proteins.pdf
- 同济大学:《细胞的生物化学》课程电子教案(课件讲稿)氨基酸代谢 Amino Acid Metabolism.pdf
- 同济大学:《细胞的生物化学》课程电子教案(课件讲稿)碳水化合物与碳水化合物代谢 Carbohydrates and Carbohydrate metabolism.pdf
- 同济大学:《细胞的生物化学》课程电子教案(课件讲稿)Aerobic Metabolism II Electron Transport and Oxidative Phosphorylation(ETC).pdf
- 同济大学:《细胞的生物化学》课程电子教案(课件讲稿)脂类代谢 Lipids and lipid metabolism.pdf
- 同济大学:《细胞的生物化学》课程电子教案(PPT课件)酶 Enzymes(主讲:王春光).pptx
- 同济大学:《细胞的生物化学》课程电子教案(PPT课件)脂代谢 Metabolism of Lipids.ppt
- 同济大学:《细胞的生物化学》课程电子教案(PPT课件)生物氧化 Biological Oxidation.pptx
- 同济大学:《细胞的生物化学》课程电子教案(PPT课件)核苷酸代谢 Metabolism of Nucleotides.ppt
- 同济大学:《细胞的生物化学》课程电子教案(PPT课件)Genetic information transfer——DNA biosynthesis(DNA replication-复制).pptx
- 北京化工大学:《生物工艺学》课程电子教案(教学大纲)生物工艺学教学大纲 Bioprocess Technology Fundamentals and Application.pdf